1 Introduction
Binary alkaline earth (AE) subnitrides AE2N (AE = Ca [1,2], Sr [3], or Ba [4]) feature a rather unique combination of ionic and metallic bonding within the same crystal structure. If one attempts to assign the typical oxidation states to the elements involved (AE2+ and N3–), one valence electron per formula unit is left over due to the requirement of the overall charge neutrality, leading to the formal description (AE2+)2N3–•e–. The crystal structure of the three binary subnitrides (space group Rm with Z = 3 in hexagonal setting, anti-CdCl2 structure type) is built from two-dimensional hexagonal double layers of AE metal atoms, with nitrogen atoms centering AE6 octahedra within the layers (Fig. 1). One would expect that the remaining valence electrons avoid the space around the formally trivalent nitride anions due to electrostatic repulsion and are thus expelled into the inter-layer region. A spatial separation of ionic and metallic bonding is the result: while ionic bonding between AE2+ and N3– holds the AE2N layers together, metallic inter-layer bonding is due to the nearly free remaining electrons, much like in metals themselves.
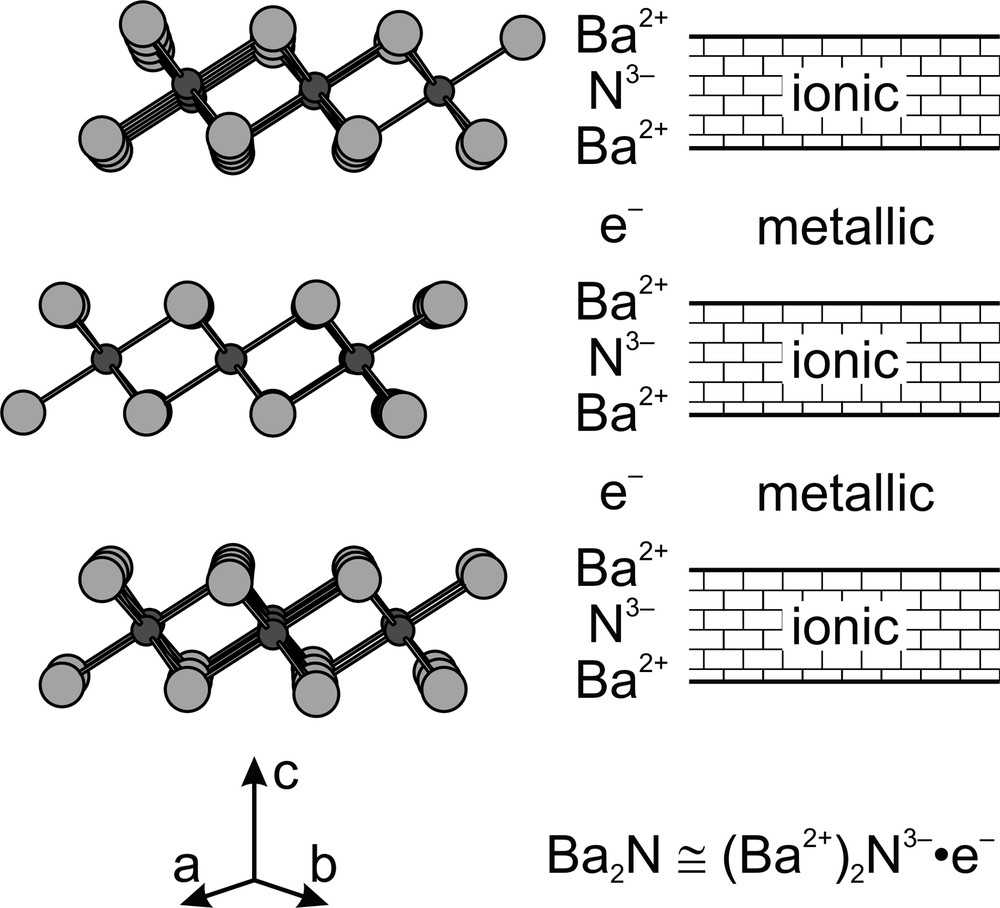
Crystal structure of Ba2N (left, space group Rm in hexagonal setting, anti-CdCl2 structure type) and formal description of chemical bonding (right). Ca2N and Sr2N are isostructural to Ba2N.
Such a bonding description, termed void metal [5], has been also postulated for the related alkali metal suboxides and other alkaline earth metal subnitrides, such as Cs11O3 and NaBa3N, for example. This viewpoint has been supported by resistivity and photoelectron spectroscopy measurements on alkali metal suboxides [6,7] and by TB-LMTO band structure calculations on NaBa3N [8]. Chemical arguments, such as bonding distances, atomic thermal displacement factors, and crystal volume considerations, also speak for the spatial separation of the two kinds of bonding in the crystal structures of suboxides and subnitrides [5,6,9]. Moreover, electrostatic repulsion between the respective anions (O2– or N3–) and the remaining valence electrons leads to a lowering of the work function, as observed in the case of Cs11O3 [7], for instance.
The interplay of ionic and metallic bonding in such compounds can be investigated further by carrying out a structural study at high-pressure: while the relatively compressible metallic regions of the respective crystal structures are expected to deform significantly under pressure, the rigid ionic regions should be much less affected by pressure. Layered AE2N subnitrides are a good test subject for such a study, as the expected anisotropy in compressibility would be apparent from the change in lattice constants under pressure, while structure refinement of the collected data would shed light on the relative compressibility of the ionic and metallic regions. Here we report the results of high-pressure powder diffraction studies, which were carried out with diamond-anvil cells (DACs) using synchrotron radiation.
2 Experimental
AE2N samples were prepared from the elements, with special attention paid to avoiding impurities such as hydrogen and oxygen: the metals were sublimed in vacuum twice and all sample handling was carried out under an atmosphere of purified argon [10]. No pressure medium was used when loading samples into DACs because of the high reactivity of the subnitrides. The ruby luminescence method was used for pressure measurement [11,12]. In some samples Ta powder was included as a pressure standard [13]. The Ca2N, Sr2N, and Ba2N samples were investigated at pressures up to 14, 30, and 40 GPa, respectively. Angle-dispersive powder X-ray diffraction patterns were measured at room temperature at the ID09 beam line of the European Synchrotron Radiation Facility in Grenoble. Monochromatic radiation with a wavelength of 0.417 Å was used for pattern collection on an image plate detector. Integration of two-dimensional images was carried out using the program FIT2D [14] yielding intensity vs. 2θ diagrams. Powder averaging was improved by rotating the DACs. Some samples were annealed at 200 °C for several hours after applying pressure, but this treatment did not significantly improve the quality of the collected diffraction patterns.
Full Rietveld refinement of the collected X-ray diagrams was carried out using the GSAS program [15]. The refined parameters were the hexagonal unit cell constants a and c, the fractional coordinate z of the alkaline earth metal atoms, isotropic thermal displacement parameters for the AE atoms (with Uiso for nitrogen fixed at 0.02 Å2), Chebyschev polynomial background, a profile function employing the microstrain broadening description of Stephens [16], a spherical harmonic preferred orientation correction necessary due to the layered nature of the compounds, phase fractions, and an overall intensity scaling factor. The Stephens function was used in order to take into account the effects of nonhydrostaticity due to the absence of a pressure medium. Further details of the crystal structure investigations may be obtained from the Fachinformationszentrum Karlsruhe, 76344 Eggenstein-Leopoldshafen, Germany, on quoting the depository numbers CSD-414329 (Ca2N-I), CSD-414330 (Sr2N-I), and CSD-414328 (Ba2N-I and Ba2N-II).
3 Results and discussion
3.1 General behavior under high pressure
The evolution of the integrated powder diffraction diagrams with increasing pressure is shown in Figs. 2–4. In the case of calcium and strontium subnitrides, pressure-induced phase transitions Ca2N-I→Ca2N-II and Sr2N-I→Sr2N-II were observed around 12 GPa. These phase transitions are reversible and the starting Ca2N-I and Sr2N-I phases were almost fully recovered upon decompression. Three pressure-induced phase transitions took place in the case of Ba2N. First, Ba2N-I transformed into Ba2N-II at 2 GPa. The next high-pressure phase, Ba2N-III, was observed only in a narrow pressure window around 7 GPa, followed by Ba2N-IV. The latter phase was also the major component observed after decompressing the sample, accompanied by a small amount of Ba2N-II.
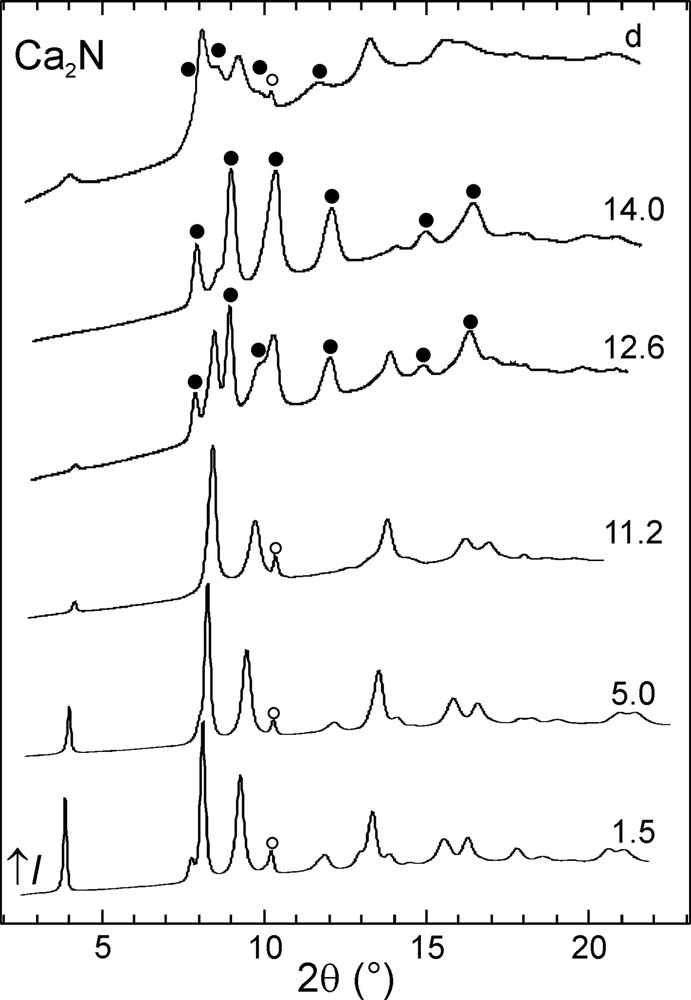
Selected powder diffraction diagrams for Ca2N. The sample pressure is given in GPa next to each pattern. The uppermost diagram was measured upon decompression. Reflections corresponding to the high-pressure Ca2N-II phase (●) and to the Ta standard (○) are marked.
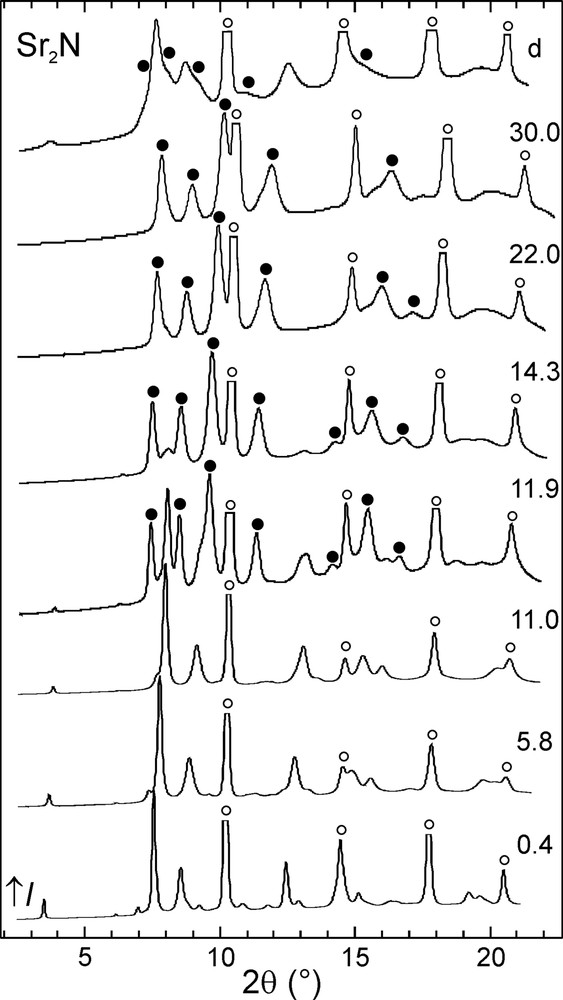
Selected powder diffraction diagrams for Sr2N. The sample pressure is given in GPa next to each pattern. The uppermost diagram was measured upon decompression. Reflections corresponding to the high-pressure Sr2N-II phase (●) and to the Ta standard (○) are marked.
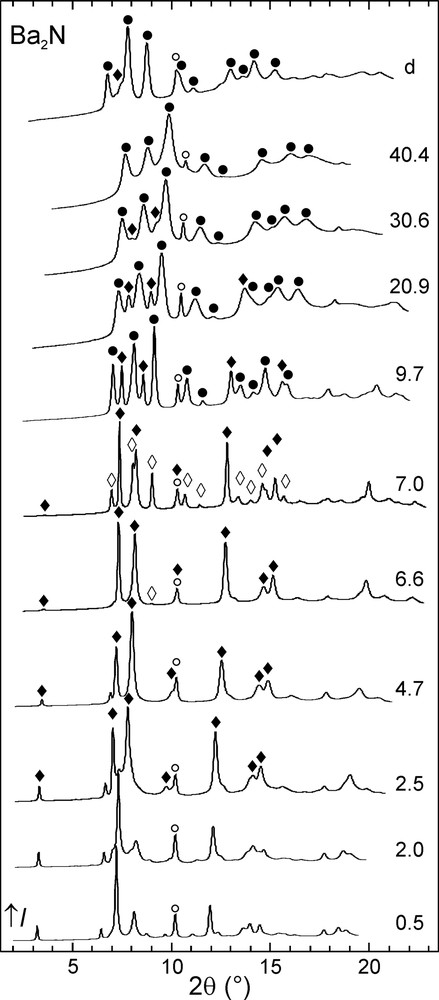
Selected powder diffraction diagrams for Ba2N. The sample pressure is given in GPa next to each pattern. The uppermost diagram was measured upon decompression. Reflections corresponding to the high-pressure Ba2N-II (♦), Ba2N-III (◊), and Ba2N-IV (●) phases and to the Ta standard (○) are marked.
The crystal structure of Ba2N-II is closely related to that of the starting phase (Ba2N-I): both consist of 2D-Ba2N layers, but the stacking of these layers is different. While the anti-CdCl2-type structure (Ba2N-I) is stable at ambient pressure, the alternative anti-CdI2 structure type (Pm1, Z = 1) is adopted by the Ba2N-II phase. Only a small volume decrease of 1.6% accompanies this transition. It has already been suggested [17], that stacking faults might play a role in the structural chemistry of the EA2N subnitrides, leading to a range of the observed values for the c lattice constant depending on the preparation conditions, for example. The existence of two energetically close ways to stack the 2D-Ba2N layers would support this suggestion. Since the qualitative bonding picture and dimensionality are preserved in this transition, we will consider Ba2N-II together with the AE2N-I phases in the following.
Full structural characterization of the high-pressure Ba2N-III, Ba2N-IV, Ca2N-II, and Sr2N-II phases was not possible based on the available diffraction data. The cubic Ba2N-III, stable only around 7 GPa, appears to have a crystal structure which is completely different from those of the layered phases. The last observed high-pressure barium subnitride phase, Ba2N-IV, as well as Ca2N-II and Sr2N-II, is likely to be a distorted variant of the cubic Ba2N-III phase. These phases will be addressed in more detail below.
3.2 Compressibility of the layered subnitrides
The collected powder diffraction patterns for the layered phases Ca2N-I, Sr2N-I, Ba2N-I, and Ba2N-II, were used to refine the unit cell parameters of the respective structures as a function of pressure. The unit cell volume per formula unit (V/Z) for the four layered structures as a function of pressure is shown in Figs. 5–7. The V(P) data were fitted using the Birch equation of state [18] in order to extract the values (all at zero pressure) of the unit cell volume (V0), bulk modulus (B0), and the first derivative of the bulk modulus (B′0). These fits resulted in the following bulk modulus values (B0): 45(4) GPa for Ca2N-I, 33(3) GPa for Sr2N-I, and 21(6) GPa for the two layered Ba2N phases. For comparison, the values of the bulk modulus for the respective metals are 17.4(1) GPa (Ca), 11.88(5) GPa (Sr), and 8.93(6) GPa (Ba) [19]. No high-pressure experimental data on the closed-shell AE3N2 nitrides exists. Only the recently reported theoretical B0 value of 70.1 GPa for Ca3N2 [20] can be taken as a reference. The experimentally measured B0 values for the ionic oxides CaO (114(9) GPa [21]), SrO (88(7) GPa [21]), and BaO (74.0(2) GPa [22]) are somewhat higher. Thus the alkaline earth metal subnitrides lie between the pure metals and the related ionic compounds as far as compressibility is concerned.
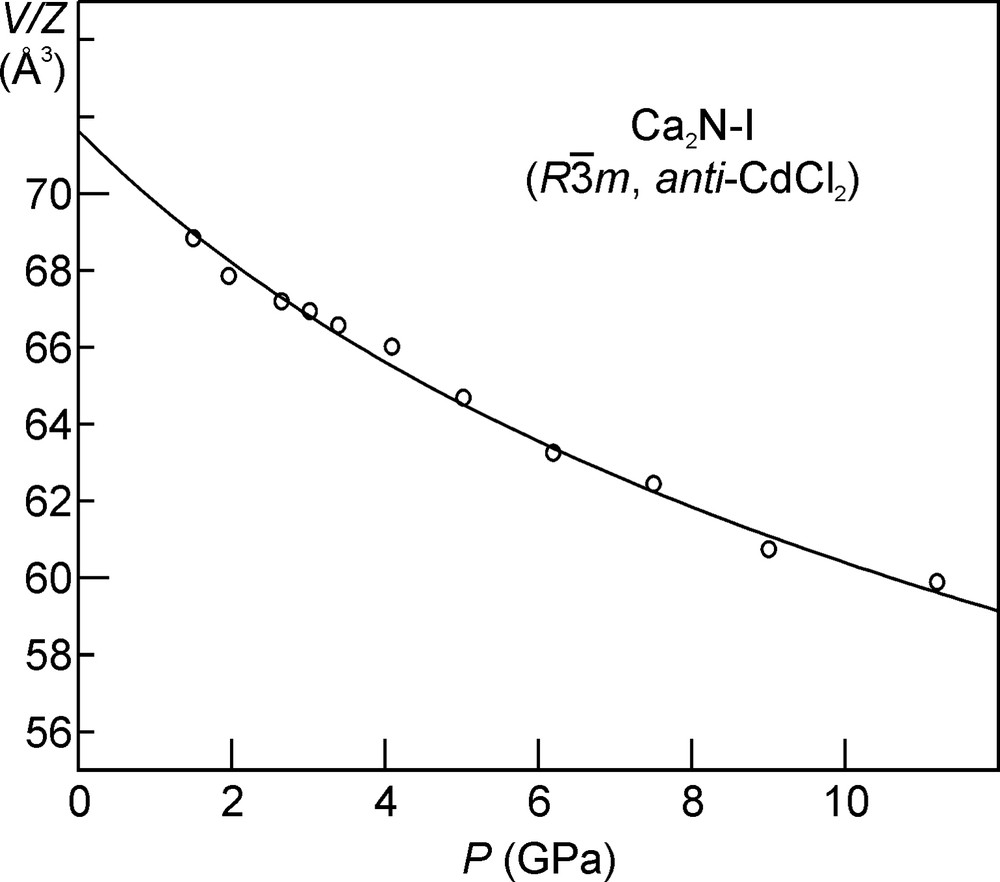
Crystal volume per formula unit as a function of pressure for Ca2N.

Crystal volume per formula unit as a function of pressure for Sr2N.

Crystal volume per formula unit as a function of pressure for Ba2N.
Anisotropic compressibility of the Ca2N-I, Sr2N-I, Ba2N-I, and Ba2N-II phases is another consequence of the layered structure. Fig. 8 shows the c/a ratio for these structures as a function of pressure. Typical for layered compounds, compressibility is larger perpendicular to the layers than in the plane of the layers, resulting in a reduction of the c/a ratio with increasing pressure. The compressibility along the c-axis was found to be about twice as large as that along the a-axis for all four phases.

The c/a ratio for the layered AE2N phases as a function of pressure. The values for the Ba2N-II phase were tripled in order to allow for a comparison. A closest packing of spheres (AE metal atoms) corresponds to the c/a value of 2√6 = 4.899.
In order to find out, whether the metallically or the ionically bonded regions of the layered subnitride phases are more compressible, Rietveld refinement of the respective crystal structures was carried out based on the diffraction patterns collected under pressure. The evolution of the only free atomic parameter (z of the AE metal atoms) as a function of pressure is shown in Fig. 9. Decreasing value of z(AE) with increasing pressure is a sign that the metallic gap between the AE2N layers is closing. In other words, the metallically bonded regions are more compressible than the ionically bonded ones. Structural details for the layered subnitrides are summarized in Table 1.
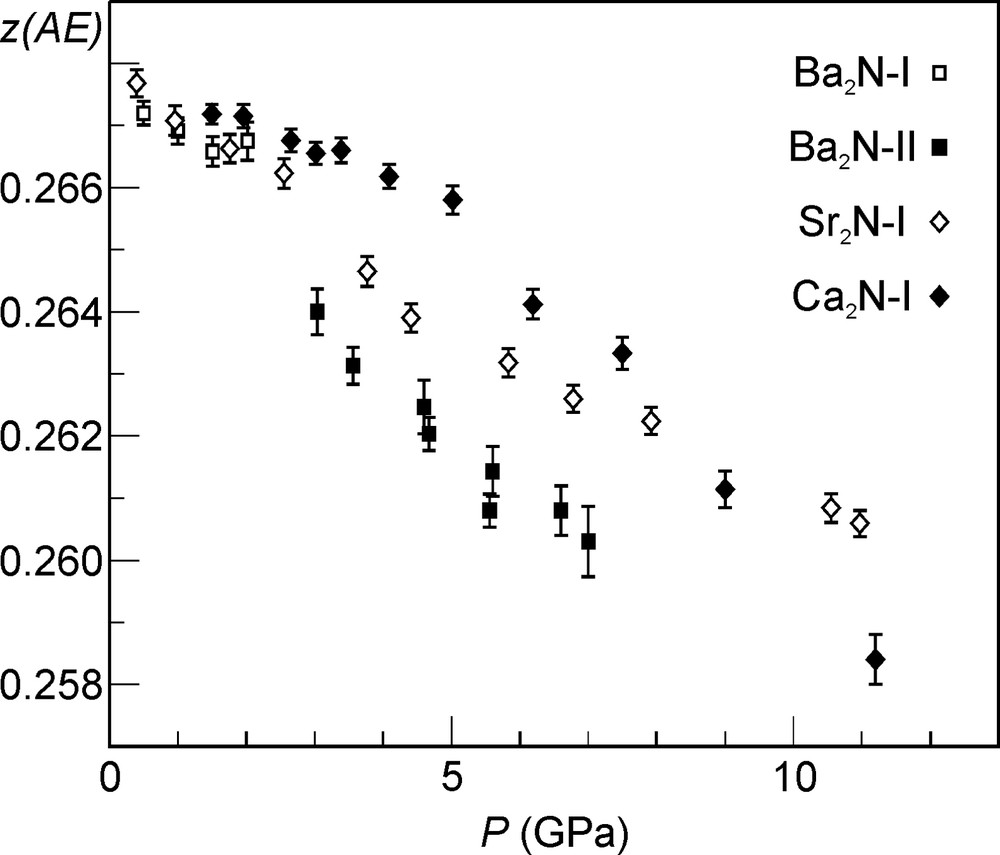
The z parameter of the AE metal atoms in the layered subnitrides as a function of pressure. The values for the Ba2N-II phase were converted according to (1 – z)/3 in order to allow for a comparison. A closest packing of spheres (AE metal atoms) corresponds to the z(AE) value of 0.25. For example, z = 0.2520 in CdCl2 [30] and (1 – z)/3 = 0.2503 in CdI2 [31].
Structural details for the layered alkaline earth-metal subnitrides
Phase | P (GPa) | Space group, Z | a (Å) | c (Å) | AE position | N position |
Ca2N-I | 4.1 | Rm, 3 | 3.5530(3) | 18.114(3) | 6c (0, 0, 0.2662(2)) | 3a (0, 0, 0) |
Sr2N-I | 4.4 | Rm, 3 | 3.7622(3) | 19.589(8) | 6c (0, 0, 0.2639(2)) | 3a (0, 0, 0) |
Ba2N-I | 1.0 | Rm, 3 | 3.9908(3) | 22.079(7) | 6c (0, 0, 0.2669(2)) | 3a (0, 0, 0) |
Ba2N-II | 4.7 | Pm1, 1 | 3.8211(3) | 6.902(2) | 2d (1/3, 2/3, 0.2138(8)) | 1a (0, 0, 0) |
3.3 Beyond the layered structures
Increase of the pressure applied to the layered subnitrides leads to phase transitions in which the 2D structural features are lost. In the case of Ba2N, a new Ba2N-III phase follows the layered Ba2N-II phase at 7 GPa. The strongest peaks of this phase could be indexed with a cubic body-centered unit cell (see also Fig. 4). Based on the observed reflection conditions the space group I3d (220) was chosen. The observed relative intensities were consistent with a crystal structure containing barium atoms in the 16c position (x, x, x).
Although this cubic phase is stable only in a narrow pressure window around 7 GPa and could therefore not be observed in a pure form, it was possible to carry out a Rietveld refinement of data collected on a Ba2N sample containing ca. 40% Ba2N-III and 60% Ba2N-II (see Fig. 10). The refined barium position with x = 0.0480(3) corresponds to the phosphorus substructure of Th3P4. The a-axis was found to be 8.3741(5) Å; refinement of the isotropic thermal displacement parameter for Ba resulted in a reasonable value of 0.026(2) Å2 for Uiso.
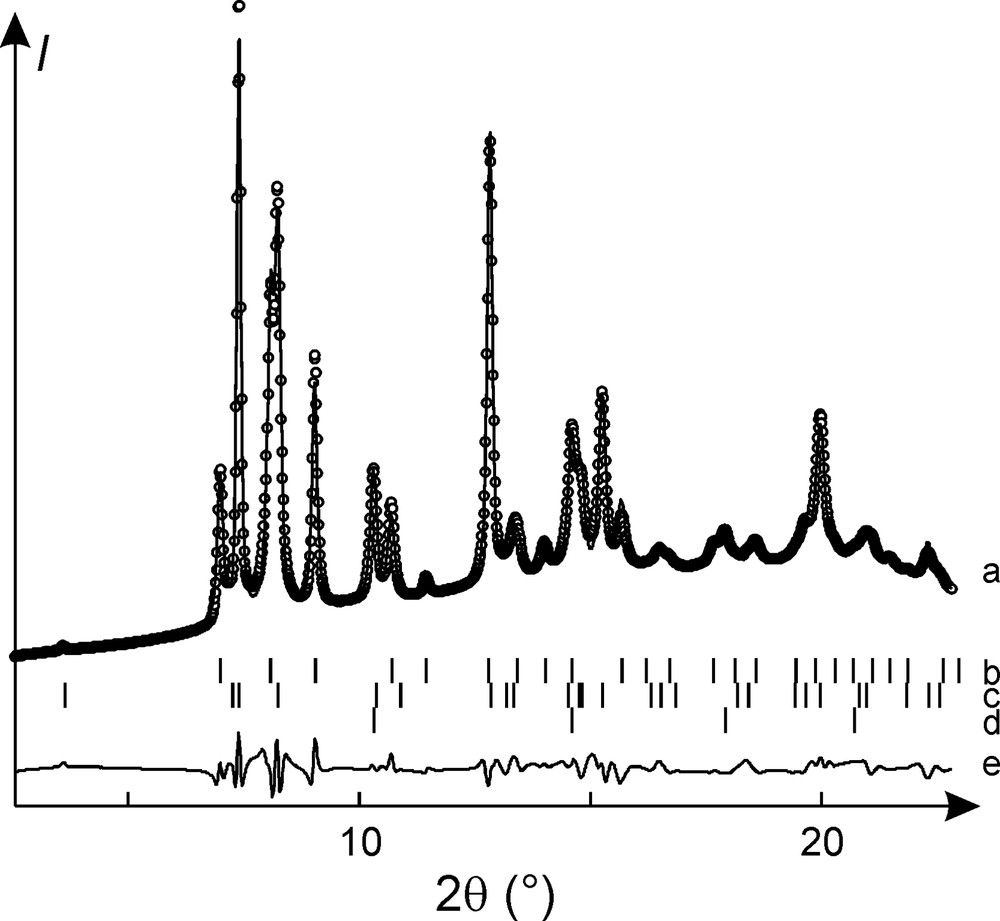
Rietveld refinement of powder diffraction data collected on a Ba2N sample at 7 GPa. The measured points are shown with circles, the fit is given by a solid line (a). The positions of the reflections for the phases involved are marked with tics (b: cubic Ba2N-III; c: layered Ba2N-II; d: Ta standard). The difference between the measured and calculated patterns is shown at the bottom (e).
The space group I3d does not have an eightfold position which would be suitable for the nitrogen atoms. Insertion of N atoms in the 12a (3/8, 0, 1/4) site (Th position in Th3P4) with the occupancy of 2/3 in order to satisfy the overall stoichiometry causes little change in the refinement results, as one might expect due to the large difference between the barium and nitrogen atoms in terms of the scattering factors.
The Ba-substructure of Ba2N-III is best described by a body-centered cubic (bcc) lattice with the unit cell doubled in all three directions and with all atoms slightly displaced from their positions along the body diagonals while maintaining the overall cubic symmetry. That is, setting the value of x to zero would return the Ba-array of Ba2N-III back to the ideal bcc arrangement as found in elemental barium at ambient conditions (see Fig. 11). The Ba-substructure of Ba2N-III can also be described as a space-filling arrangement of tetrahedra and bisdisphenoids (trigon-dodecahedra). The 12a sites lie exactly in the centers of these bisdisphenoids. If these positions were to be occupied by the nitrogen atoms, the coordination number of the latter would increase from six in the layered subnitrides to eight. Assuming this crystal structure for Ba2N-III, a crystal volume decrease of 8% accompanies the Ba2N-II→Ba2N-III phase transition.

Distortion of a bcc arrangement of Ba atoms leading to the Ba-substructure of the cubic Ba2N-III phase. Thick lines represent the edges of the resulting tetrahedra and bisdisphenoids, while the thin lines track the bcc lattice.
Although we are not aware of other examples of Th3P4-type structures with a partial occupation of the Th-site, there are quite a few reports on A2BX4 compounds with A and B atoms taking this position. While in some cases no deviation from the cubic symmetry was observed (as in an anti-type Rb4Cl2O [23], see also references therein), ordering of the different atoms occupying the Th-position can lead to a lowering of symmetry as in K2MIICl4 (MII = Sr, Ba, Eu, or Pb), for example [24,25].
The partial occupation of the 12a position by the nitrogen atoms might be the reason for a relatively low structural stability of the cubic Ba2N-III phase, which exists in a narrow pressure window. One might expect, that still higher pressure induces an ordering of the N atoms in two thirds of the available bisdisphenoids, leading to a less symmetrical structure. Indeed, such a distortion apparently takes place and a new phase, Ba2N-IV, results. Although the details of its structure are still unknown, the overall shape of its powder diffraction pattern resembles that of the cubic Ba2N-III with clear signs of a distortion, such as splitting of the existing and appearance of new peaks. Interestingly, in the cases of Ca2N and Sr2N direct transitions from the anti-CdCl2-type structures (Ca2N-I and Sr2N-I) to the distorted anti-Th3P4-type structures (Ca2N-II and Sr2N-II) take place. The analogy between the latter two structures and the Ba2N-IV phase can be assumed based on the similarities between their powder diffraction patterns (compare Figs. 2–4).
It is remarkable that a cubic cI16 (the Ba-substructure of Ba2N-III) structure was recently observed in high-pressure studies on elemental Li [26]. This cubic phase was found to be superconducting with Tc between 10 and 20 K according to different reports [27–29].
4 Conclusions
The high-pressure behavior of the layered AE2N (AE = Ca, Sr, or Ba) subnitrides was investigated up to 40 GPa by means of powder X-ray diffraction using a synchrotron radiation source. A new layered modification of the barium subnitride, Ba2N-II with the anti-CdI2-type structure, was observed in the pressure range from 2 to 7 GPa. The observed bulk modulus values for the layered subnitrides are larger than those for the pure metals, but lower than those for the related ionic compounds. The compressibility along the c-axis was found to be about twice as high compared to that along the a-axis for all layered AE2N phases, resulting in a decreasing c/a ratio with increasing pressure. The z parameter of the AE metal atoms was also found to decrease under pressure, indicating higher compressibility of the metallically bonded inter-layer region in the AE2N structures. All of these findings are in agreement with the chemical bonding picture for these compounds according to the formal description (AE2+)2N3–·e–. Further increase of pressure was found to induce phase transitions resulting in a cubic anti-Th3P4-type Ba2N-III structure, related to a high-pressure modification of Li, and its distorted variants Ca2N-II, Sr2N-II, and Ba2N-IV.
Acknowledgments
Thanks are due to the ESRF, Grenoble, for making its facilities available for this investigation (project CH-1115). G.V.V. would also like to thank the Alexander von Humboldt Foundation, the Federal Ministry of Education and Research, and the Programme for Investment in the Future (ZIP) of the German Government for financial support.