1 Introduction
Since the pioneering work of Fyfe [1] and Eckert [2] in the 90 s, 1H–X–Y triple resonance solid-state NMR probes are now used in the frame of inorganic materials, as invaluable tools of structural investigation. Various {X–Y} pairs have been considered, including the most frequently used, {27Al,31P}, {27Al,29Si} and {19F,29Si}. Demonstration of the use of more ‘exotic’ {X,Y} pairs has been also proposed in the literature, including: {31P,113Cd} [2b], {27Al,13C} [3], {31P,77Se} [4], {31P,29Si} [5]. The NMR techniques used so far can be roughly divided in three categories:
- • (i) CP MAS [6,7] experiments (cross polarization magic angle spinning), involving either a single X→Y transfer or a double 1H→X→Y transfer (with Y detection in both cases). In the latter case, 1H irradiation is mainly used for T1 purposes;
- • (ii) REDOR [8] (rotational-echo double resonance) and TEDOR [9] (transferred-echo double resonance) experiments;
- • (iii) J-mediated techniques, like J-resolved and HMQC [10] (heteronuclear multiple quantum coherence) experiments.
The pulse sequences described in (i) and (ii) rely on the dipolar interaction between X and Y nuclei and allow to establish spatial connectivities by 1D (CP, REDOR, TEDOR) or 2D (CP HETCOR, heteronuclear correlation) [1,11] experiments. In some cases, distances between nuclei were even accurately measured by studying carefully the dipolar oscillations of the variable contact time CP curves or by REDOR experiments [1f,12–14]. The spectroscopic approach cited in (iii) establishes connectivities between X and Y nuclei through the isotropic J coupling, and allows the direct proof of chemical bonding between X and Y [15].
The main goal of this article is to highlight double X/Y and triple 1H/X/Y CP MAS experiments, involving ‘exotic’ spin pairs such as X/Y = 51V/29Si; 31P/13C; 29Si/13C. One notes that in all cases, at least one resonant nucleus presents a low natural abundance (29Si: 4.7%; 13C: 1.1%). In the 1H/29Si/13C CP MAS experiments, both target nuclei can be considered as low abundant nuclei. The results presented here are definitely encouraging for the set-up of 2D correlation experiments (such as HETCOR CP MAS experiments). Moreover, three compounds were found to act as good candidates for the set-up of the Hartmann–Hahn condition under MAS, namely: C6H5PH(O)(OH) for the 31P/13C transfer [SiO1.5(C2H3)]8 for the 29Si/13C transfer and [AsW9O33(tBuSiO)3(VO)]3– for the 51V/29Si transfer. Such experiments seem interesting for the fine description of polyoxometalate derivatives and silica/phosphate based hybrid materials [16–19].
2 Experimental
A typical triple resonance MAS experiment used in this work is presented in Fig. 1. The acquisition of the Y spectra is performed under 1H CW or TPPM decoupling and with/or without X high-power CW decoupling.
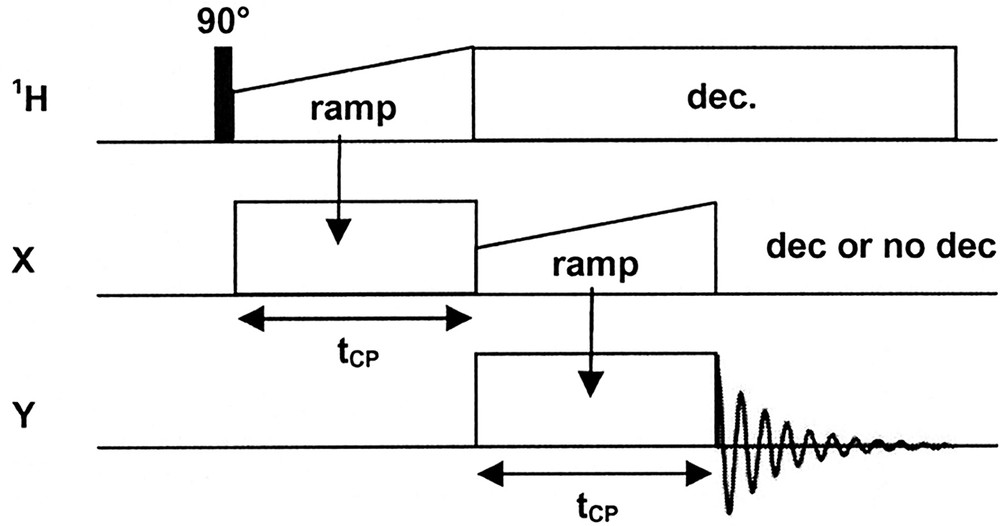
Typical triple resonance experiment used in this work (under MAS). X = 31P, 29Si; Y = 13C, 29Si. CW decoupling on the X channel (29Si, 31P, 51V) is optional (see text for details).
1H/51V/29Si experiments were performed on a Bruker AVANCE 400 spectrometer (51V: 105.40 MHz; 29Si: 79.48 MHz). Zirconia rotors (4 mm) were spun at the magic angle at 2 and 5 kHz (51V coupled and 51V decoupled, respectively). The polyoxometalate (POM) derivative [AsW9O33(tBuSiO)3(VO)]3– ((nBu4N)+ salt) (Fig. 2) was used as a standard. The multistep synthesis of this hybrid compound proceeds as follows: (i) synthesis of the trivacant anion [AsW9O33]9– by co-condensation of WO42– and AsO2– under aqueous acidic conditions, (ii) reaction with tertiobutyltrichlorosilane tBuSiCl3 to afford the organosilyl open-structure hybrid [AsW9O33(tBuSiOH)3–] as [nBu4N]+ salt [24], and (iii) reaction with vanadyltrichloride VOCl3 leading to closing-up of the structure by the capping group VO3+ [25]. Standard 1H/29Si CP MAS experiment (including 1H CW high-power decoupling) was combined with CW 51V decoupling. As 51V is a quadrupolar nucleus, the CW power level on the 51V channel was carefully adjusted, in order to obtain the best resolution on the 29Si spectra. Indeed, it is now well known [11] that efficient decoupling from quadrupolar nuclei is best obtained at rather low power levels (here 30 kHz on the 51V channel). Other decoupling schemes (such as TPPM) were not used in this case. Chemical shifts are referenced towards TMS (0 ppm). Typical parameters were: recycle delay: 5 s, contact time (1H→29Si): 5 ms; number of scans (NS) = 4380 for the fully coupled 29Si spectrum (7-mm CP MAS Bruker probe: standard 29Si CP MAS spectrum; 4-mm triple resonance CP MAS Bruker probe: 51V decoupled 29Si CP MAS spectrum).
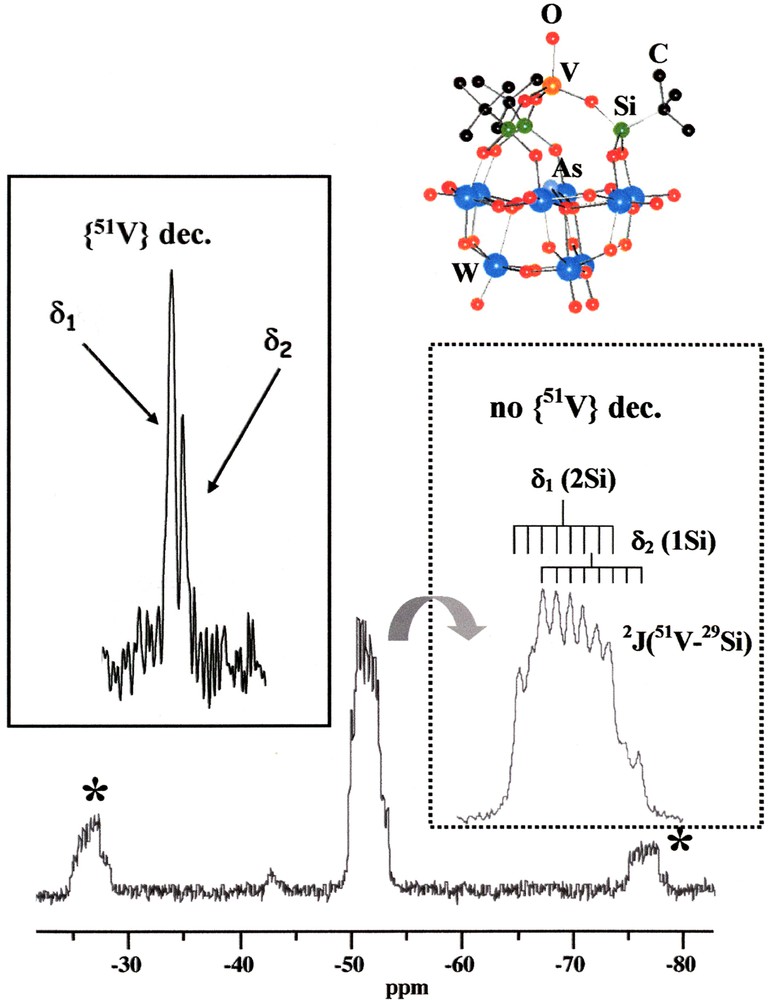
29Si CP MAS spectrum (2 kHz) of [AsW9O33(tBuSiO)3(VO)]3– and the corresponding ORTEP view (orange: V, gray: As, blue: W, red: O, black: C, green: Si). Dashed inset: isotropic lines without {51V} decoupling. Full line inset: isotropic lines with CW {51V} decoupling (MAS at 5 kHz, ★ spinning sidebands).
1H/31P/13C and standard 1H/13C CP MAS experiments were performed on a Bruker AVANCE 300 spectrometer (4-mm triple resonance CP MAS Bruker probe) (13C: 75.43 MHz; 31P: 121.44 MHz). Zirconia rotors (4 mm) were spun at the magic angle at 5 kHz. The phenylphosphinic acid C6H5PH(O)(OH) (purchased from Fluka) was used as a standard compound (see Fig. 3). For the 1H/13C CP MAS experiment: recycle delay: 20 s; contact time (1H→13C): 2 ms; NS: 832. For the 1H/31P/13C CP MAS experiment: recycle delay: 20 s; contact time (1H→31P): 2 ms; contact time (31P→13C): 5 ms; NS = 2500. In both cases, variable-amplitude cross-polarization (VACP) pulse schemes were used [20]. In particular, a VACP scheme is absolutely necessary for the second CP transfer (31P→13C), as the rather weak 31P–13C heteronuclear dipolar coupling is strongly modulated by the MAS process. The second contact time (31P→13C) is adapted for the detection of close neighbors of the 31P nuclei. This parameter can be used for spectral editing purposes. The 13C acquisition was performed under 1H TPPM decoupling, but without 31P decoupling (the TPPM parameters – phase and pulse duration – were adjusted by using the phenylphosphinic acid). Chemical shifts were referenced towards TMS (0 ppm) via solid adamantane.
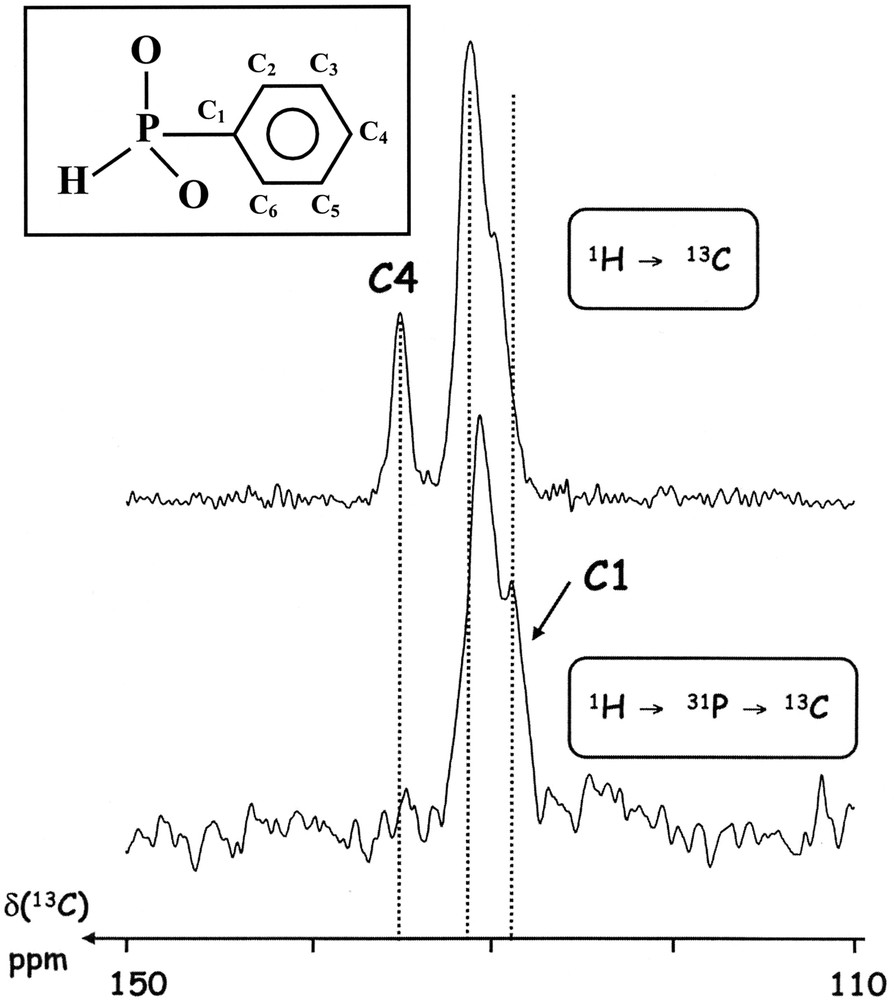
1H/13C and 1H/31P/13C CP MAS spectra of the phenylphosphinic acid C6H5PO(OH)H (see the inset for the C atoms labeling scheme).
The 1H/29Si/13C experiments were performed on a Bruker AVANCE 400 spectrometer (4-mm triple resonance CP MAS Bruker probe) (13C: 100.56 MHz; 29Si: 79.48 MHz). Zirconia rotors (4 mm) were spun at the magic angle at 5 kHz. The octavinylsilsesquioxane [SiO1.5(C2H3)]8 derivative (Fig. 4) (purchased from Hybrid Plastics) was used as a standard for the set-up of the experiment. The following parameters were used: recycle delay: 15 s; contact time (1H→29Si): 10 ms; contact time (29Si→13C): 5 ms, NS = 15000. TPPM decoupling on the 1H channel was used during the 13C acquisition but 29Si decoupling was not performed.
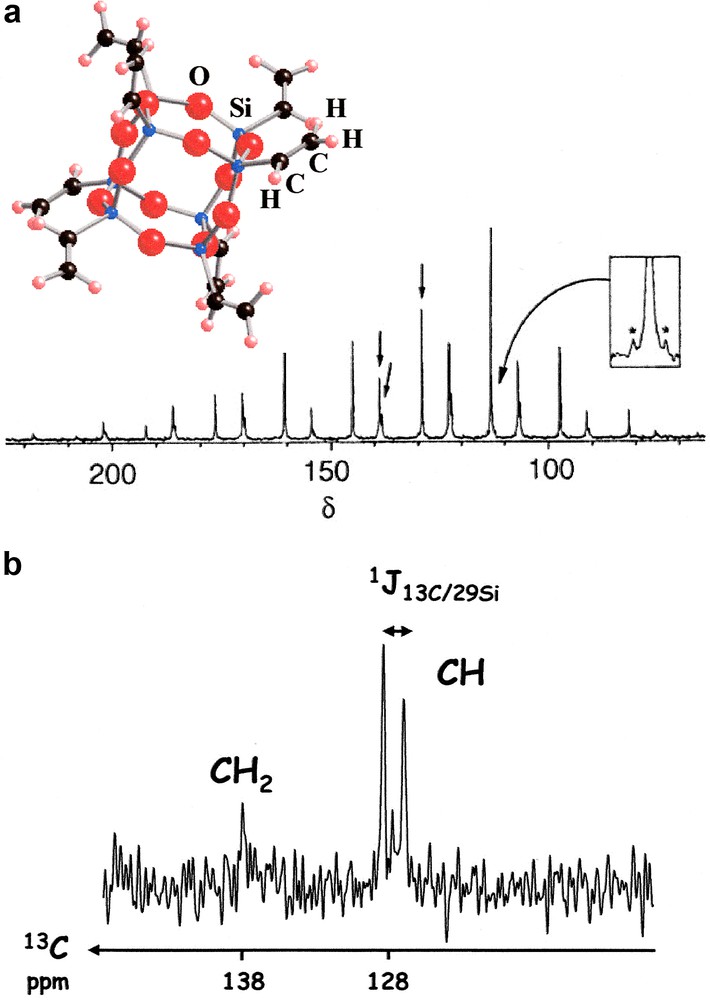
(a) Standard 1H/13C CP MAS spectrum of [SiO1.5(C2H3)]8 at low spinning rate and the corresponding ORTEP view (blue: Si, red: O, black: C, white: H). Isotropic lines are shown by arrows. Inset: *satellites related to the 13CH resonance due to 1J(13C–29Si) coupling. (CH2: δ ≈ 138 ppm, CH: δ = 128 ppm). (b) 1H/29Si/13C spectrum. The splitting due to 1J(13C–29Si) is clearly observable.
3 Results and discussion
3.1 1H/29Si experiments with and without {51V} decoupling
The 1H→29Si CP MAS spectrum (at 2 kHz) of [AsW9O33(tBuSiO)3(VO)](nBu4N)3 is presented in Fig. 2. This compound is one member of the large polyoxometalate family [16]. The polyoxometalate core ‘AsW9O33’ is capped by a vanadium atom linked through three tBuSiO groups (see the ORTEP representation in Fig. 2). This entity can be considered as intermediate between organic and inorganic moieties and acts, therefore, as a hybrid nanocluster [18]. The ORTEP view shows that at most three 29Si isotropic resonances are expected. The 29Si CP MAS spectrum (without {51V} decoupling) exhibits a complex isotropic line, involving at least 10 resonances. Such a line can be decomposed into two isotropic contributions (δ1 –52.3 ppm, 2Si; δ2 –53.0 ppm, 1Si) subjected to the isotropic J coupling interaction (2J51V–29Si = 28 Hz). As I = 7/2 for 51V, eight lines are expected for each 29Si resonance. Whereas the three Si atoms are equivalent in solution, leading to a unique 29Si resonance, this is no longer the case in the solid state, where the three Si atoms are crystallographically independent. The differences in the electronic surrounding of these three nuclei appear not sufficient to resolve three resonances.
By using CW {51V} decoupling during the 29Si acquisition (at an adequate RF power – see Section 2), the spectrum is clearly simplified. All splitting due to 2J51V–29Si couplings are efficiently suppressed and only two isotropic 29Si resonances are observed (δ1, δ2). These are in the ratio 2:1, as expected from the fully coupled spectrum. The high-frequency signal is, however, significantly broader, likely because the two crystallographically unequivalent silicon nuclei are not strictly isochronous. Therefore, it is shown that triple resonance experiments involving exotic pairs, such as 51V/29Si, open new areas in the structural studies of polyoxometalate derivatives.
3.2 1H / 31P / 13C experiments on the phenylphosphinic acid: towards 31P / 13C spectral editing
The phenylphosphinic acid C6H5PH(O)(OH) (see Fig. 3) appears as a good candidate for the set-up of the 31P/13C Hartmann–Hahn condition under MAS. Indeed, such a compound is characterized by a direct P–H bond, leading to a very efficient 1H/31P CP transfer. Moreover, the attached phenyl group exhibits 13C nuclei, which can be easily distinguished through their distances to the 31P nucleus. The quaternary C1 atom is directly related to P, whereas the C4 (para) atom is characterized by the longest P···C distance. It is, therefore, expected that the CP experiment (based on the dipolar coupling) will allow a clear distinction between 13C nuclei, based on the strong variation of 31P–13C distances. We note, however, that the T1(1H) relaxation processes at ν0 = 300 MHz (B0 = 7 T) are rather long, leading to a relaxation delay of 20 s (see Section 2). To the best of our knowledge, spectral editing in the frame of 31P/13C NMR spectroscopy in natural abundance was never proposed in the literature, though some data involving fully labeled 31P/13C spin pairs were published by Hagaman [21].
The standard 1H/13C CP MAS spectrum is presented in Fig. 3. It is characterized by strong resonances located at δ ≈ 130 ppm and related to the phenyl groups. One resonance (δ = 135.1 ppm) is clearly deshielded and can be safely assigned to the C4 atom [22].
By using the double CP transfer 1H/31P/13C, the 13C{1H} spectrum is strongly modified (31P/13C contact time: 5 ms). The line centered at δ = 135.1 ppm is no more observed, in agreement with the proposed assignment (C4). Moreover, the part of the spectrum centered at δ = 129.2 ppm is overestimated under the 31P/13C CP transfer. This line can be assigned to C1. However, the 13C acquisition was performed under 1H decoupling, but without 31P high-power decoupling. It has been shown previously that 1J31P–13C in phenyl derivatives can be estimated to ~200 Hz [22]. We believe that the line at δ = 129.2 ppm corresponds to one branch of the C1 doublet, due to the isotropic 1J31P–13C splitting. The second branch of the doublet is surely located at δ ≈ 130.9 ppm, superimposed to (C2,C6) resonances.
Further experiments, involving {31P} high-power decoupling and variable 31P/13C contact time, will be necessary for the complete assignment of the 13C spectrum. Such experiments are now in progress in the laboratory.
3.3 1H / 29Si / 13C experiments in natural abundance
The octavinylsilsesquioxane [SiO1.5(C2H3)]8 (Fig. 4) has been carefully studied by 1H/29Si and 1H/13C CP MAS experiments [23]. It can be considered as a starting building block for the synthesis of hybrid material exhibiting tailored porosity [19]. The standard CP 1H/13C experiment shows resonances assigned to 13CH2 and 13CH groups, in good agreement with crystallographic data [23]. The 13CH resonance is characterized by satellites due to 1J13C–29Si splitting. This isotropic J coupling was estimated to |1J13C–29Si| ≈ 136 Hz. The double CP (1H/29Si/13C) experiment is presented in Fig. 4b. The signal-to-noise (S/N) ratio is rather poor (NS = 15000 – see Section 2), as 13C and 29Si are present in natural abundance. However, this spectrum is highly informative and proves that 1H/29Si/13C experiments can be performed on hybrid silica materials. The 13CH2 resonances are strongly underestimated, as expected. Indeed, CH2 groups are characterized by long Si···C distances (2.75 Å), when compared to the CH groups (1.81 Å). Moreover, the 13CH resonance is splitted into a doublet, corresponding to 1J13C–29Si coupling, as no {29Si} high power decoupling was applied during 13C acquisition. One notes that the intrinsic S/N ratio can be easily improved by using {29Si} high-power decoupling.
4 Conclusion
Three examples, involving rather ‘exotic’ spin pairs 51V/29Si, 31P/13C and 29Si/13C, have shown that triple resonance experiments are particularly adequate for the characterization of inorganic and hybrid molecules/materials. These examples were based on double CP transfer under MAS conditions in 1D version. 2D HETCOR versions and J-derivated experiments [15] can be surely performed as well. The phenylphosphinic acid C6H5PH(O)(OH), the polyoxometalate derivative [AsW9O33(tBuSiO)3(VO)]3– and the octavinylsilsesquioxane [SiO1.5(C2H3)]8 can act as potential standards for the set up of the Hartmann–Hahn condition in the frame of 31P/13C, 51V/29Si and 29Si/13C CP MAS NMR, respectively.