1 Introduction
New materials based on electrochemically active polymers are continuously being developed for numerous applications [1–3]. They are used as solid electrolytes in batteries [2–4], as electrocatalysts [5–7], and as ion sensors [8,9]. They can be also used in electronic nanodevices [10–12]. Polymeric materials used in these fields of science and technology should exhibit redox activity. From this point of view, fullerene-based polymers are very attractive. Up to six, reversible, one-electron steps are observed for the reduction of C60 in solution [13]. At relatively high potentials, a single one-electron oxidation is observed for C60 [14]. Fullerene cages incorporated into polymeric structures are expected to retain this sort of electrochemical activity.
Structures of polymers containing fullerenes are shown in Fig. 1. Like alkenes, fullerenes can form homopolymers through (2 + 2) cycloaddition. Formation of these structures can be induced through excitation by photons [15–17], electrons [18], in plasma discharge [19], and as a result of hydrostatic pressure [20,21]. Fullerene homopolymers are relatively unstable and therefore have limited practical application.
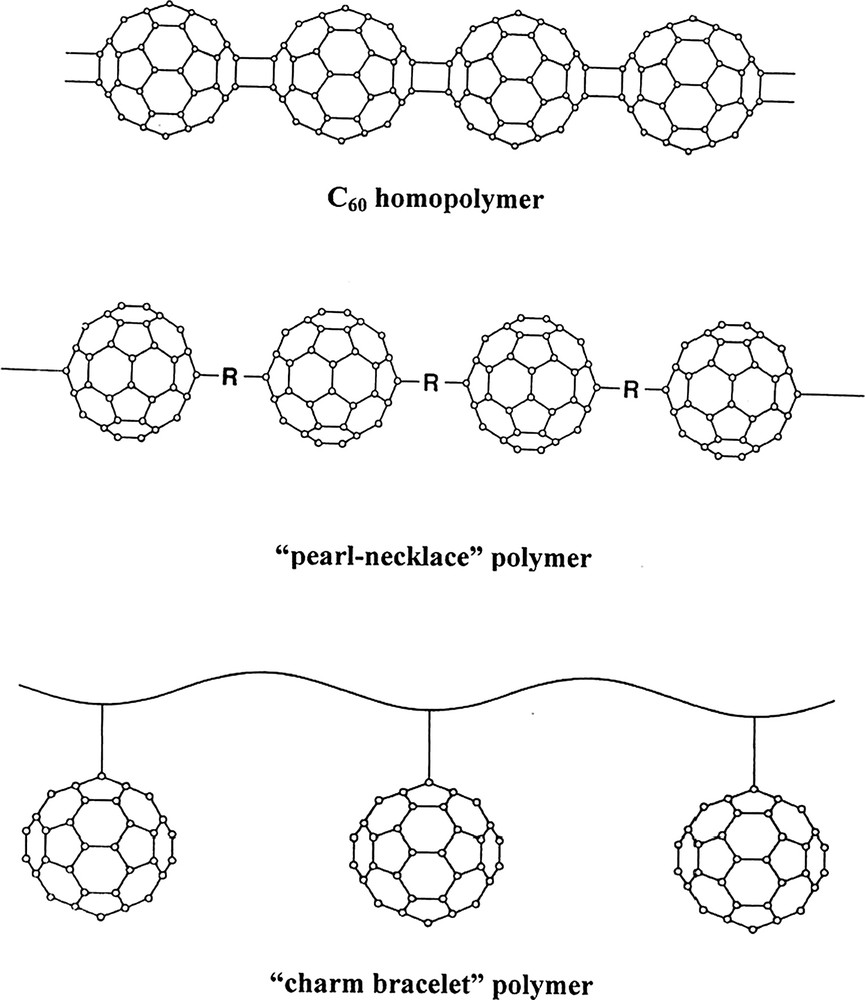
Structures of fullerene polymers.
Incorporation of fullerene moieties into other polymeric structure is also possible [22]. This incorporation may be accomplished in two general ways. The in-chain addition results in formation of ‘pearl-necklace’ like polymers. They are highly cross-linked and insoluble in most solvents. The C60–p-xylene copolymer is an example of such structure [23].
The C60 molecules can also be introduced into the side chain of a polymer as a pendant group to form ‘charm-bracelet’ polymers. There are two approaches to the formation of such polymers: the reaction of a fullerene or fullerene derivative with preformed polymer or polymerization of monomer containing a fullerene as a pendant functional group. The main advantage of ‘charm-bracelet’ polymers is their well-defined, stereochemically controllable arrangement of the fullerene moieties. The C60-on-chain polymers can be prepared by titration of toluene solution of C60 with aminopolymers, such as poly(ethylene imine) or poly(propylene imine) [24]. They can be also formed via the reaction of fullerenes with carbanion intermediates of polystyrene, poly(p-bromostyrene) or poly(vinylbenzylchloride) [25,26]. Linear polymers containing azide functional groups, such as azidomethyl-substituted polystyrene, react with C60 to form ‘charm-bracelet’ C60-polystyrene polymers [27]. C60 modified poly(N-vinylcarbazole) and its bromine- and iodine-modified derivatives exhibit photoconductive properties [28,29].
Polymers can also be doped with C60 without the formation of covalent bonds between the fullerene and the polymeric chains. For example, polyvinylcarbazole [30] and poly[2-methoxy,5-(2′-ethylhexyloxy)-p-phenylene vinylene] [31] have been doped with fullerenes (C60 or C70). The photoconductivities of these materials are comparable with some of the best photoconductors available commercially.
Electrochemical methods were also used for forming fullerene-based polymers and their immobilization on the electrode surfaces. These techniques allow control of the amount of polymer deposited, its morphology, and its oxidation state. Electrochemically produced polymeric systems can be divided into four groups:
- ● ‘charm-bracelet’ polymers formed from the electrochemically active fullerene derivatives;
- ● ionically induced polymers;
- ● fullerene epoxide-based polymers;
- ● ‘pearl-necklace’ co-polymers of fullerenes and transition-metal complexes.
The ‘charm-bracelet’ polymers are formed from fullerene derivative precursors. The oxidation of alkylthiophene [32–34] or alkylsilbutadienyl [35] groups initiate the polymerization process. The fullerene groups attached to the resulting polymeric chains retain their electrochemical properties.
The galvanostatic electrolysis carried out in solution containing C60 and LiClO4 results in formation of C60 homopolymer via ionically induced (2 + 2) cycloaddition [36].
An electrochemical procedure for the formation of redox-active films with the epoxide C60O as the precursor has been developed [37,38]. It has been postulated that the electrochemically formed C60O2- anion initiates the polymerization process [38]. In the polymeric network, C60 cages may be connected through covalent C–O–C linkages, although direct C–C linkages are also likely to be involved. Related films are readily prepared by the reduction of C60 and C70 in the presence of limited amounts of dioxygen in a toluene/acetonitrile mixture [39,40].
A two-component electroreductive process that involves the reduction of C60 (or a C60 derivative) in the presence of transition-metal complexes of Pt, Pd, Ir, and Rh has been developed [41–44]. The fullerene/transition-metal films that result are particularly interesting. In these systems, the polymeric network is believed to be formed through covalent bonding between transition-metal atoms (or complexes) and fullerenes.
This review covers the electrochemical formation and properties of fullerene/transition-metal films. Attention is also paid to electrochemical studies of the formation and properties of such films containing C60 derivatives with covalently attached electron-donating groups. The potential applications of these redox-active, fullerene/transition-metal-containing polymers are discussed.
2 Electrochemically induced polymerization of fullerenes and transition-metals
Electrochemical reduction of solutions of C60 and certain transition-metal complexes leads to the formation of dark colored, redox-active films on the electrode surface (Figs. 2 and 3). Table 1 lists some of the transition-metal complexes that have been used as precursors for film formation. The C60/Pd and C60/Pt films obtained from reduction of C60 and palladium(II) complexes (e.g. palladium(II) acetate or Pd(benzonitrile)2Cl2) or platinum(II) complexes (e.g. cis-Pt(py)2Cl2 or {Pt(μ-Cl)Cl(C2H4)}2) have been studied the most intensively [41,42,45–49]. In both systems, the fullerene moieties are believed to be bonded to the metal atoms in η2 fashion to form extended chains. Additional platinum or palladium atoms can form cross-links between these chains. Fig. 2 shows the cyclic voltammograms involved in the formation of the C60/Pd film [42]. Trace (a) shows the cyclic voltammetry of C60 alone, while trace (b) shows the corresponding data for Pd(benzonitrile)2Cl2. As seen in trace (c) upon repeated scanning of potential an increase of the current in the range of fullerene reduction is observed when a mixture of C60 and Pd(benzonitrile)2Cl2 is subject to multiscan cyclic voltammetry. During the process a new electroactive phase is formed on the electrode surface. The reduction of the palladium complex to form a metallic phase initiates polymerization. Similar behavior was observed for the process of C60/Pt film formation [41]. The following mechanism was proposed for the process of C60/Pd and C60/Pt electropolymerization [50].
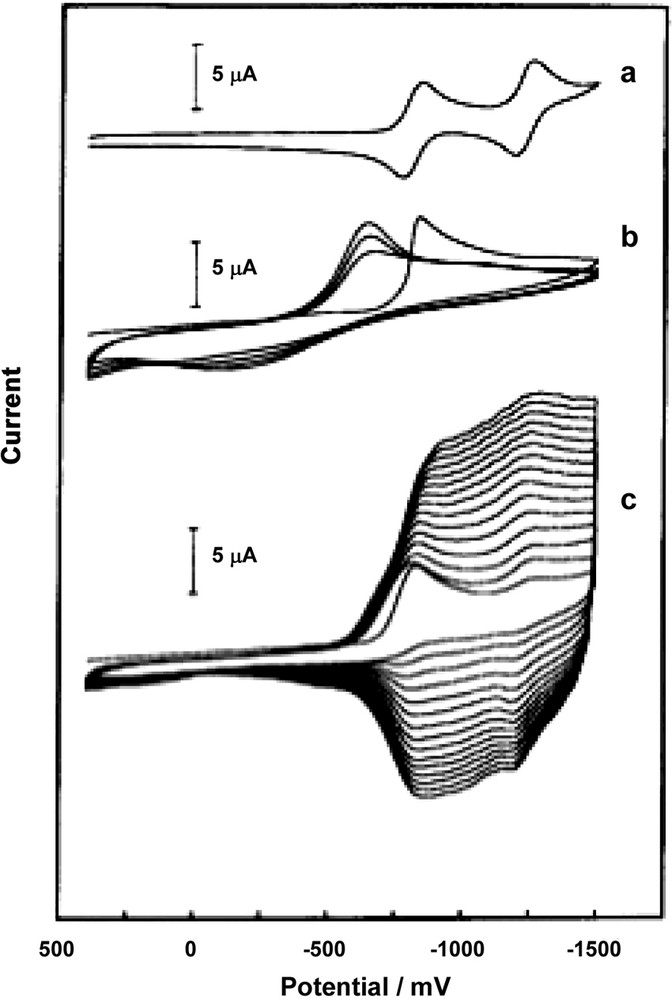
Multicyclic voltammograms obtained for: (a) 0.65 mM C60, (b) 0.50 mM (PhCN)2PdCl2, and (c) 0.30 mM C60 and 0.50 mM (PhCN)2PdCl2 in an acetonitrile/toluene (1:4. v/v) solution containing 0.10 M tetra(n-butyl)ammonium perchlorate at an Au (1.5-mm diameter) electrode. The sweep rate was 100 mV/s [41].
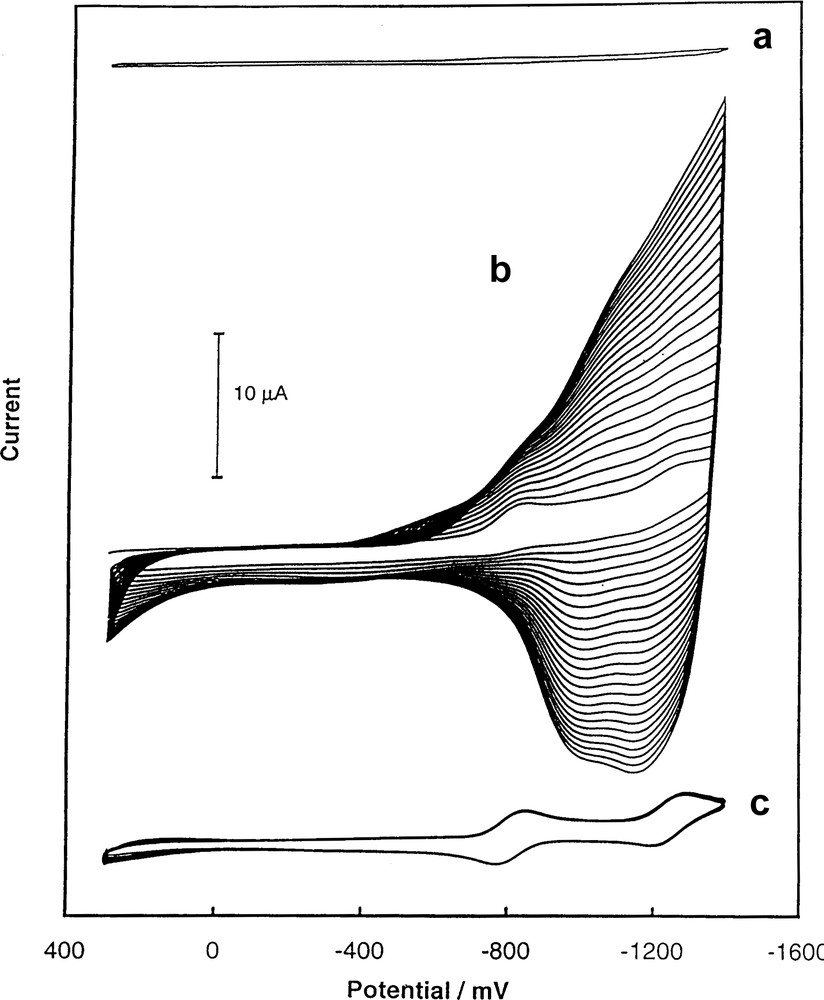
(a) Cyclic voltammogram obtained for a 0.9 mM solution of (CF3CO2)4Rh2. (b) Multicyclic voltammograms (24 cycles) for a solution of 0.25 mM C60 and 0.85 mM (CF3CO2)4Rh2. (c) Multicyclic voltammograms (20 cycles) from a solution containing 0.25 mM C60, 0.85 mM (CF3CO2)4Rh2, and 60 mM pyridine. Data were gathered at a gold electrode in acetonitrile/toluene mixture (4:1) containing 0.10 M tetra(n-butyl)ammonium perchlorate. The sweep rate was 100 mV/s [41].
Precursors for electropolymerization to form C60/M
Polymer of C60 and transition-metal | Transition-metal complex precursors | |
C60/Pd | [Pd(CH3CO2)2]3 | Pd(CH3CO2)2 |
Pd(PhCN)2Cl2 | trans-PdCl2(py)2 | |
C60/Pt | cis-PtCl2(py)2 | trans-PtCl2(py)2 |
PtI2(py)2 | [Pt(μ-Cl)Cl(C2H4)]2 | |
C60/Rh | [Rh(CO)2Cl2]2 | Rh(1,5-COD)2SO3CF3 |
[Rh(CF3CO2)]2 | RhCl3(py)3 | |
C60/Ir | [IrCl(cyclooctane)2]2 | Ir(CO)2Cl(p-toluidine) |
C60/Au | AuCl(AsPh3) | (CH3)2SAuCl |
C60/Ag | Ag(CH3CO2) |
Electroreduction of the Pt(II) or Pd(II) complexes and formation of metal zero-valent intermediates, M0–C60, initiates the growth of the C60/M film on the electrode surface (path A in Scheme 1) with the second polymer growth stage involving many individual steps. Simultaneously, the deposition of a metallic palladium or platinum phase may take place (path B). The relative amount of the two products depends on the ratio of concentration of the Pd(II) (or Pt(II)) complex to that of the fullerene in solution. High ratios are needed to obtain deposition of the metallic phase.
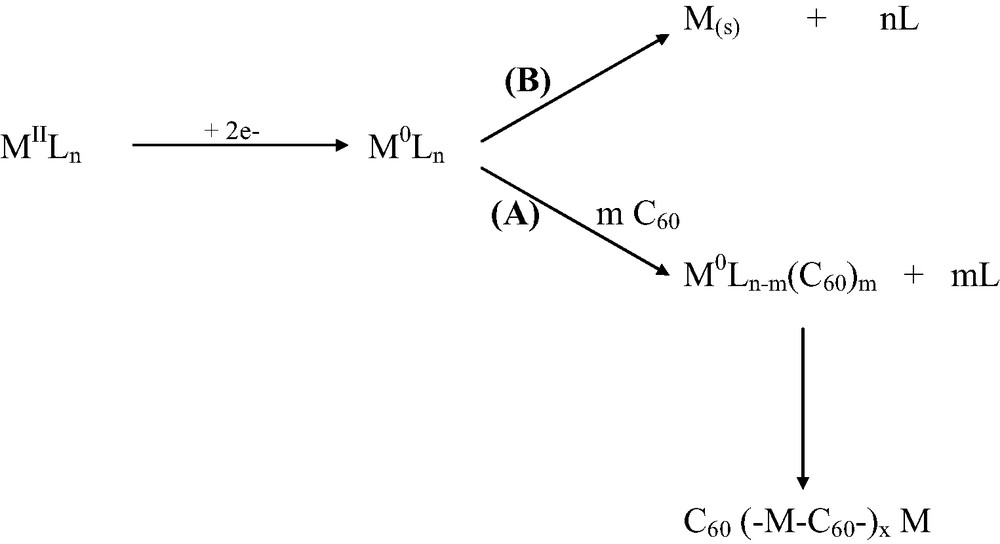
The electrochemically formed C60/Pd and C60/Pt films are related to the materials prepared chemically by the reaction of C60 with palladium(0) or platinum(0) complexes – Pd2(dba)3 or Pt(dba)2 (where dba is dibenzylideneacetone) [51–54]. Depending on the reaction stoichiometry, the composition of these materials is C60/Mn (M = Pt and Pd) where n ranges from 1 to 7. A schematic illustration for the formation and structure of these polymers is shown in Fig. 4 [51]. Both the chemically prepared and the electrochemically formed C60/Pd and C60/Pt polymers react with phosphines to produce monomeric complexes of the type (η2-C60)M(PPh3)2 [41,54]. These monomers have been crystallographically characterized, and the ability to extract the (η2-C60)M unit from these polymers by reactions with ligands constitutes significant evidence for the presence of direct covalent interactions between the metal atoms and the fullerenes in these polymeric materials.
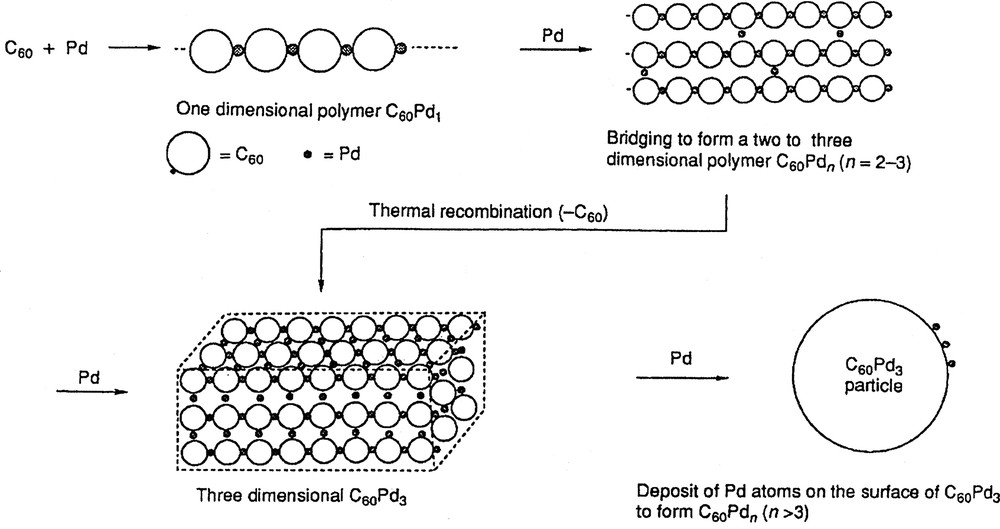
Proposed mechanism for the chemical formation of the C60/Pd polymers [51].
In the films prepared by reduction of C60 in the presence of either Rh2(CF3CO2)4 or Ir(CO)2Cl(p-toluidine), the fullerene molecules are apparently bridged by Rh2(CF3CO2)4 or Ir(CO)2 moieties. These films are also formed at the negative potentials needed for C60 reduction. However, the iridium or rhodium complexes used as precursors for polymerization are electrochemically inactive in this potential range. The cyclic voltammograms involved in the formation of the C60/Rh film are shown in Fig. 3 [41]. In this film, fullerene cages are apparently axially coordinated to the rhodium dimer Rh2(CF3CO2)4. Pyridine, which competes for occupation of the axial coordination sites, inhibits film growth as seen in trace (c) of Fig. 3. In the case of the C60/Ir film, Ir(CO)2 units bridge fullerene moieties and the infrared spectrum of the film shows ν(CO) at 2035 and 1970 per cm [41].
3 Electrochemical properties of C60/Pd and C60/Pt films
Films prepared from C60 or C70 and certain transition-metal complexes exhibit electrochemical activity in the negative potential range due to the reduction of fullerene moieties. Fig. 5 shows the voltammetric response of these films after transfer to acetonitrile solution containing only tera(n-butyl)ammonium perchlorate [41]. The C60/Pd film exhibits the most reversible voltammetric behavior. The process of the film reduction at negative potentials is accompanied by the transport of charge-compensating counter ions within the polymer matrix. The doping of the film by cations can be described by the following equation:
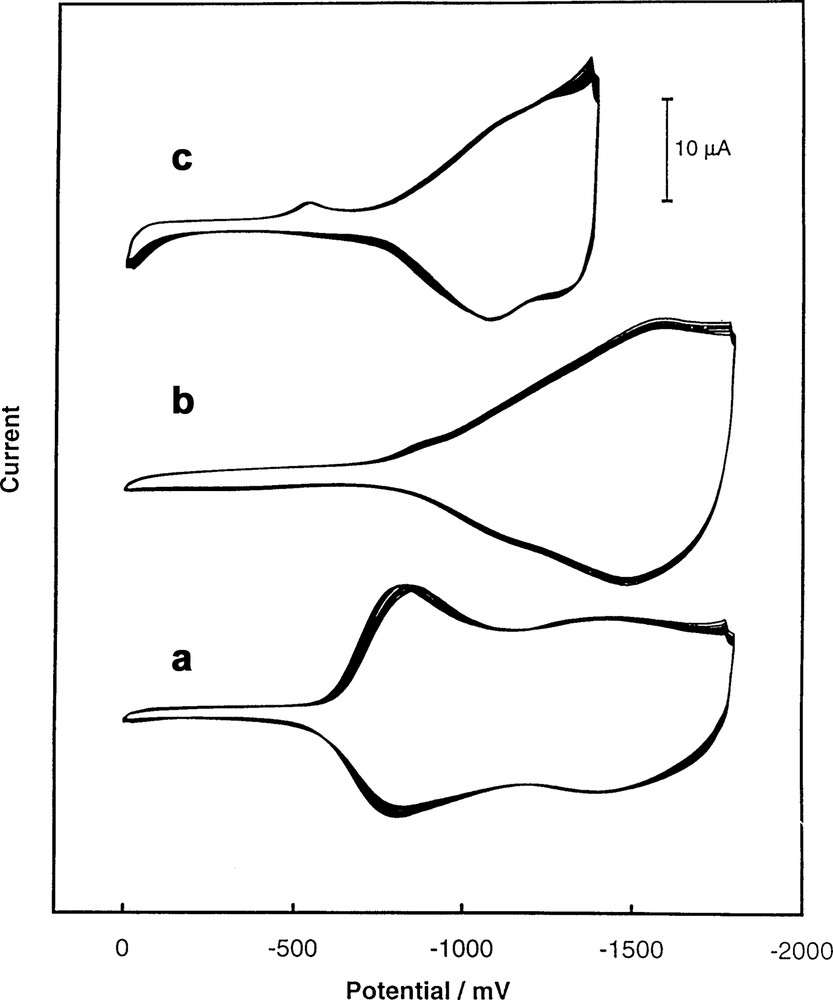
Multicyclic voltammograms of electropolymerized films obtained from (a) (PhCN)2PdCl2/C60, (b) Ir(CO)2Cl(p-toluidine)/C60, and (c) (CF3CO2)4Rh2/C60 in acetonitrile containing 0.10 M tetra(n-butyl)ammonium perchlorate with no electroactive solute at an Au (1.5-mm diameter) electrode. The sweep rate was 100 mV/s [41].
Cations of supporting electrolyte, Cat+, are transferred from the bulk of the electrolyte into the reduced chain sites. For C60/M films, the transport of counter ions within the polymer matrix controls the overall charge percolation. Therefore the electrochemical properties of these polymers are affected by:
- ● the morphology of polymeric layer;
- ● the nature of the supporting electrolyte;
- ● the solvent.
Fig. 6 shows the morphology of the C60/M (M = Pd, Ir and Rh) films electrochemically deposited on the gold foil. The transition-metal precursor significantly influences the structure of the film. A relatively flat surface with few outcroppings was observed for both the C60/Pd and the C60/Ir films. The C60/Rh and C60/Pt films are much more porous and considerably rougher. The C60/Pt layer consists of the spherical outcroppings.
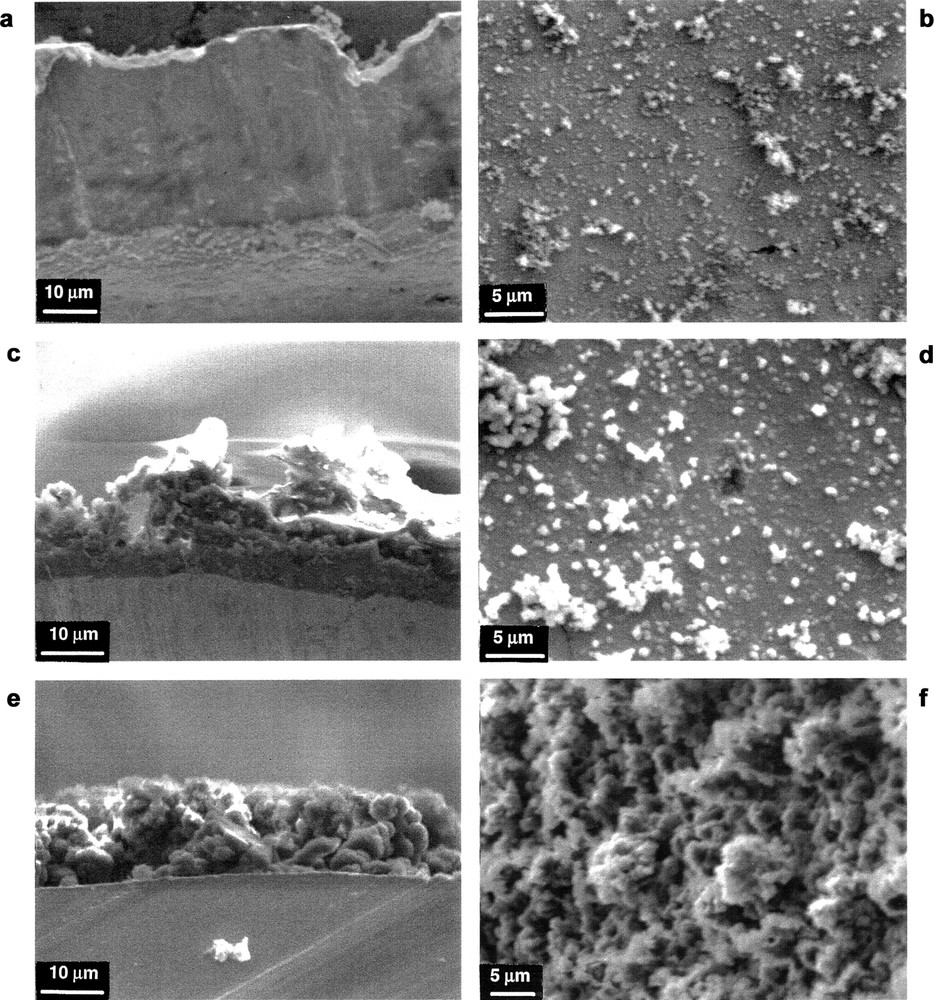
Scanning electron microscopic images of electropolymerized films from (a and b) (PhCN)2PdCl2/C60, (c and d) Ir(CO)2Cl(p-toluidine)/C60, and (e and f) (CF3CO2)4Rh2/C60. Views a, c and e are cross-sections of the film with the platinum electrode at the bottom. Views b, d and f look down at the surface of the films [41].
Fig. 7 shows the effect of the supporting electrolyte on the voltammetric behavior of the C60/Pd film. The charge involved in the reduction and re-oxidation of the film increases with a decrease in the size of cation. Small cations can migrate more easily through the pores of the polymer. The resistance of the film also depends on the nature of the specific cation of the supporting electrolyte. Fig. 8 shows the dependence of resistance of the C60/Pd film on the reduction potential for different supporting electrolytes. The experimental setup for those measurements is shown in Fig. 8a. Two sets of microelectrode arrays were covered by the C60/Pd film. When the polymer is deposited in sufficient amount, the microelectrode arrays can be connected in the electrical sense. Current can be passed between connected microelectrodes when there is an applied potential Vd between them. The magnitude of the current depends on the potential Vd and the specific resistance of the C60/Pd layer. Fig. 8c shows that the resistance of the film decreases in the potential range of the film reduction. The magnitude of the current flowing through the film (id) depends of the applied potential Vd. In the potential range in which the fullerene redox centers in the C60/Pd network are neutral, the polymer is highly resistive. The reduction of the film at negative potentials results in a significant increase of the conductivity of the layer. The strong effect of supporting electrolyte on the resistance of the C60/Pd film is also observed (Fig. 8d). The rate of charge transfer through the layer depends on the size of counter ions transport during the film reduction.
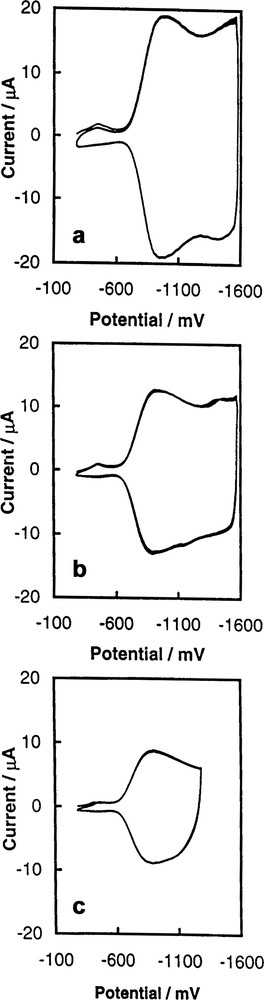
Multicyclic voltammograms of the C60/Pd film electropolymerized from [Pd(CH3CO2)2]3 and C60 in acetonitrile containing (a) 0.10 M tetra(ethyl)ammonium perchlorate, (b) 0.10 M tetra(n-butyl)ammonium perchlorate, and (c) 0.10 M tetra(n-hexyl)ammonium perchlorate at an Au (1.5 mm diameter) electrode. The sweep rate was 100 mV/s [45].
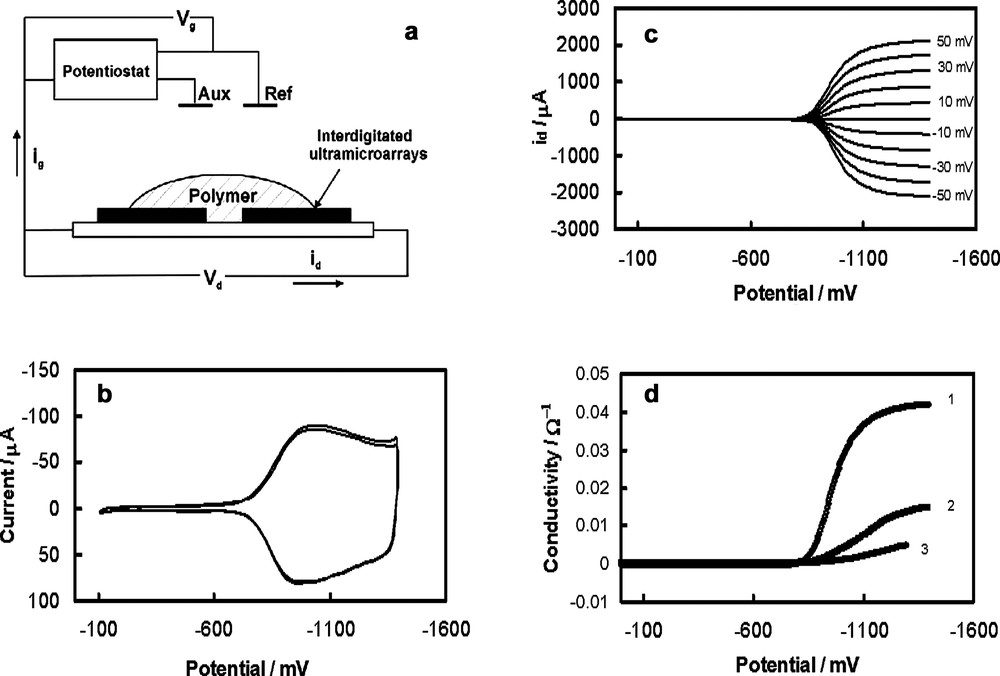
(a) A schematic picture of the system used for conductivity measurements. (b) Cyclic voltammogram of the C60/Pd film electropolymerized from Pd(CH3CO2)2 and C60 in acetonitrile containing 0.10 M tetra(n-butyl)ammonium perchlorate at interdigitated arrays electrode. (c) Dependence of the id current of the C60/Pd film electropolymerized from Pd(CH3CO2)2 and C60 on the potential obtained with platinum interdigitated microelectrode arrays in acetonitrile containing 0.10 M tetra(n-butyl)ammonium perchlorate for different Vd potentials (values of the Vd potential are shown on the figure). (d) Dependence of the resistance of the C60/Pd film electropolymerized from Pd(CH3CO2)2 and C60 on the potential obtained with platinum interdigitated microelectrode arrays in acetonitrile containing 0.10 M tetra(ethyl)ammonium perchlorate (curve 1), 0.10 M tetra(n-butyl)ammonium perchlorate (curve 2), and 0.10 M tetra(n-hexyl)ammonium perchlorate (curve 3).
The conductivity properties of these C60/M (M = Pd and Pt) films affect the electron transfer processes of redox-active species dissolved in solution [48,49]. Fig. 9 shows the voltammetric behavior of decamethylferrocene and cobaltocenium hexafluorophosphate on a bare electrode and on an electrode covered with C60/Pd film. In the latter case, if the formal potential of the additional redox system is located in the potential range in which the fullerene redox centers in the C60/Pd network are neutral, as is the case with decamethylferrocene, then electron transfer to the added component is inhibited. However, the electron can be transferred reversibly through the reduced C60/M film, if the added redox component has a formal potential with the potential range where the fullerene centers of the film are reduced. Such is the case when cobaltocenium hexafluorophosphate is added.
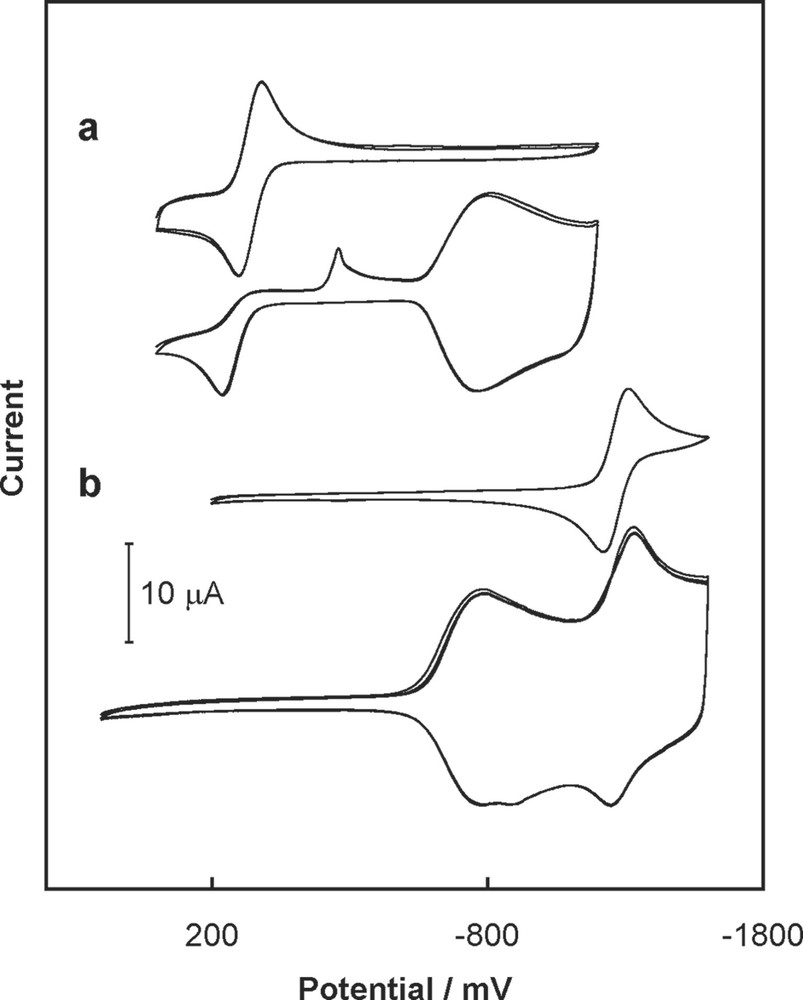
Cyclic voltammogram recorded in acetonitrile containing 0.10 M tetra(n-butyl)ammonium perchlorate and (a) 0.70 mM ferrocene and (b) 0.60 mM cobaltocenium hexafluorophosphate at Au (1.5-mm diameter) bare electrode and an electrode covered with film electropolymerized from Pd(CH3CO2)2 and C60. The sweep rate was 100 mV/s [48,49].
The C60/M films are essentially insoluble, but are stable in both aprotic and protic solvents. Solvent molecules penetrate the structure of these polymeric films. The degree of solvent swelling influences the dielectric properties of the C60/M films. Consequently, the voltammetric response of the film depends of the solvent. The C60/Pd film exhibits reversible electrochemical behavior in acetonitrile, dichloroethane, N,N-dimethylformamide and acetone solutions containing only a supporting electrolyte [45]. In contrast, voltammograms for the C60/Pd film obtained in benzonitrile, o-dichlorobenzene, and particularly dimethyl sulfoxide and propylene carbonate reveal that the electrochemical reduction of the film is rather slow [45].
4 Influence of metallic particles on the structure and properties of C60/Pd and C60/Pt films
The structure of the C60/M film formed on the electrode surface depends on the composition of the growth solution. For the C60/Pd and C60/Pt films, simultaneous deposition of the C60/M polymer and of metallic particles of palladium or platinum takes place. The relative amount of the metallic particles in the film increases with an increasing concentration of the transition-metal complex precursor in the growth solution. Fig. 10 shows a high-resolution transmission electron microscope image and selected area diffraction pattern for the C60/Pd film formed in a solution containing a high concentration of palladium(II) acetate [48]. A cubic crystalline phase of palladium metal is present in the film. The sample exhibits lattice planes with a 0.22-nm inter-planar distance and a mean palladium grain size of 4–8 nm.
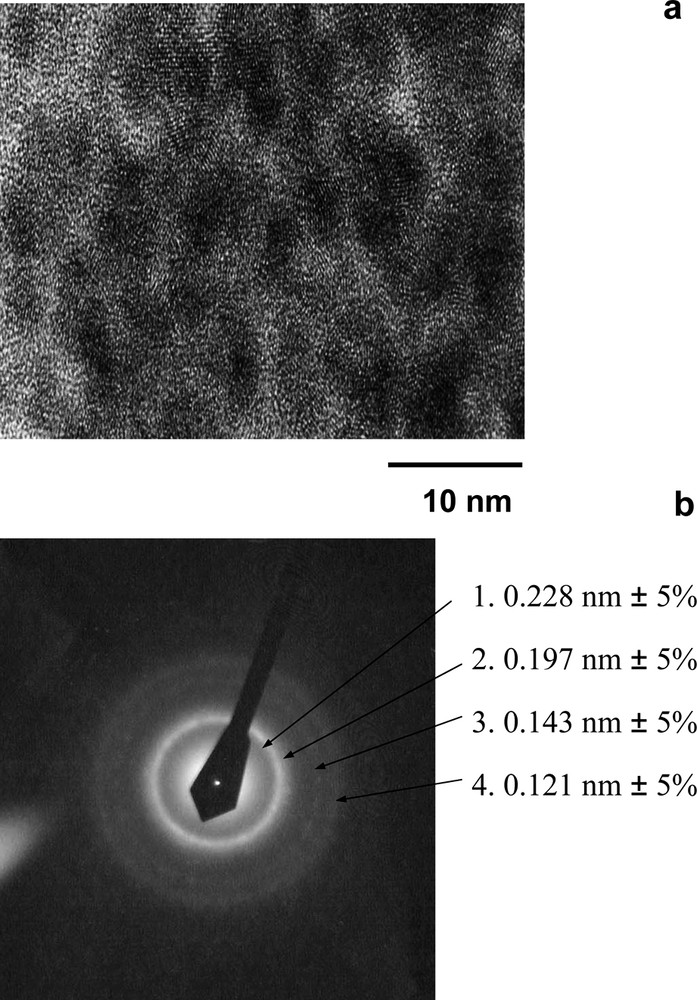
(a) High resolution transmission electron microscopy image and (b) selected area diffraction pattern of the C60/Pd film electropolymerized on the gold foil under cyclic voltammetry conditions in acetonitrile/toluene (1:4, v/v) containing 0.25 mM C60, 1.25 mM [Pd(CH3CO2)2]3, and 0.10 M tetra(n-butyl)ammonium perchlorate [48].
In Fig. 11, the effect of different ratios of the concentration of the precursors, [Pd(acetate)2]3 and C60, on the morphology of C60/Pd films formed under cyclic voltammetry conditions is shown [48]. With a high ratio, a relatively smooth film of uniform thickness is grown. A decrease in the amount of the palladium complex in the growth solution results in the formation of a rough, porous surface. Similar results were obtained for the process of C60/Pt film formation [42].
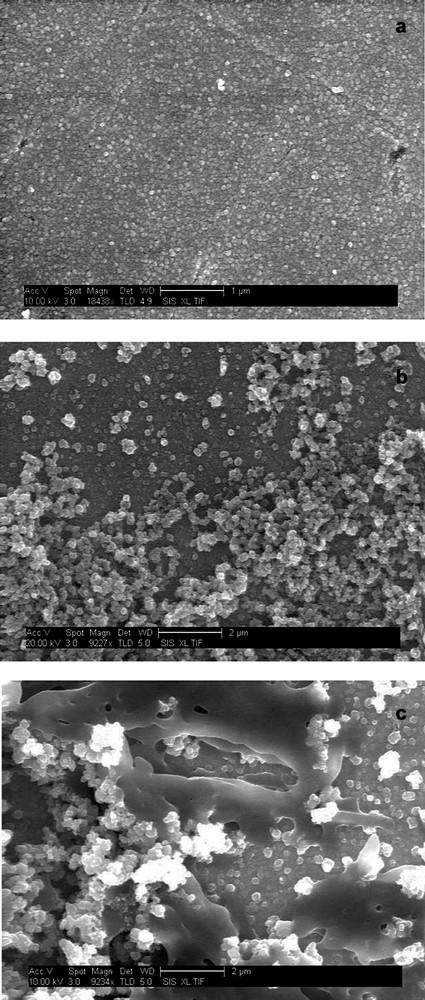
Scanning electron microscopy images of the C60/Pd film electropolymerized on the gold foil under cyclic voltammetry conditions in acetonitrile/toluene (1:4, v/v) containing 0.10 M tetra(n-butyl)ammonium perchlorate, 0.25 mM C60, and (a) 1.48 mM [Pd(CH3CO2)2]3, (b) 0.80 mM [Pd(CH3CO2)2]3, and (c) 0.41 mM [Pd(CH3CO2)2]3 [48].
The electrochemical properties of the C60/M (M = Pd and Pt) films are also influenced by the presence of metallic particles [46]. These particles can participate in the charge transport through the film. The transport of electrons during the film reduction is accompanied by the transport of counter-ions from the solution to the solid phase. Both processes are affected by the structure of the C60/Pd film in the opposite manner. The polymeric (–C60–Pd–)n chains present in the film electropolymerized in solution with relatively large excess of palladium complexes are more likely short and separated by Pd nanoclusters. The compact and non-porous structure of this film limits the rate of counter-ion transport during the film reduction. In this case, irreversible voltammetric behavior is observed for this film after it has been transferred to a solution containing only the supporting electrolyte (Fig. 12a) [46].
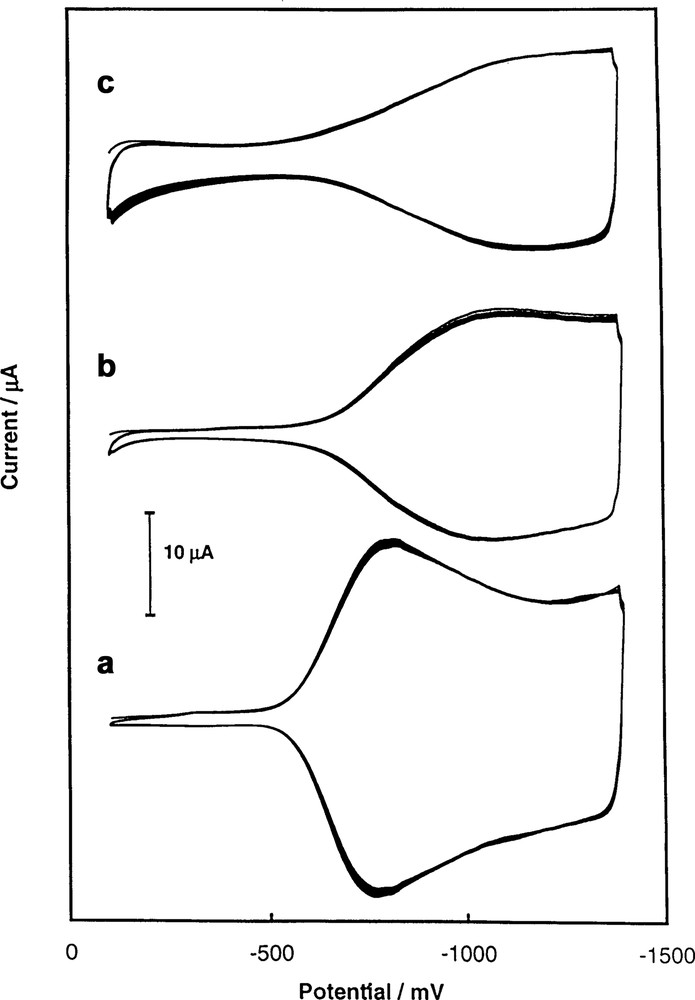
Multicyclic voltammograms of films electropolymerized from [Pd(CH3CO2)2]3 and C60 in acetonitrile containing (a) 0.10 M tetra(n-butyl)ammonium perchlorate. The sweep rate was 100 mV/s. The C60/Pd films were grown at an Au (1.5 mm diameter) electrode under cyclic voltammetry conditions in acetonitrile/toluene (1:4, v/v) containing 0.10 M tetra(n-butyl)ammonium perchlorate, 0.31 mM C60, and (a) 0.45 mM [Pd(CH3CO2)2]3, (b) 0.86 mM [Pd(CH3CO2)2]3, and (c) 1.48 mM [Pd(CH3CO2)2]3 [46].
In contrast, films formed in solutions containing a low concentration of the palladium complexes are much more porous. The structure of these films allows for solvent wetting and for penetration of the film by cations of the supporting electrolyte. Reversible voltammetric response is observed for such a film in acetonitrile containing supporting electrolyte as seen in Fig. 12c [46].
The metallic palladium or platinum nanoparticles can effectively participate in electron transfer processes. In Fig. 13, the dependences of the conductivity of films formed in solution with different ratios of Pd(acetate)2 to C60 on the potential are shown. Films formed from a solution with a low concentration of the palladium complex exhibit lower conductivity at potentials less negative that the potentials of film reduction. In this potential range, the electron transfer processes of electroactive solutes present in the solution are inhibited [49]. The resistance of the film drops in the potential range of film reduction at potentials more negative than –800 mV. The changes of resistance are related to electroreduction of fullerene moieties and film doping with counter-ions of the supporting electrolyte. If the amount of metallic nanoparticles in the film increases, the film also becomes conductive in the potential range less negative that the potential required for film reduction. The electron transfer processes for the redox-active species dissolved in solution are not inhibited by the film containing large amount of metallic palladium nanoparticles [48].
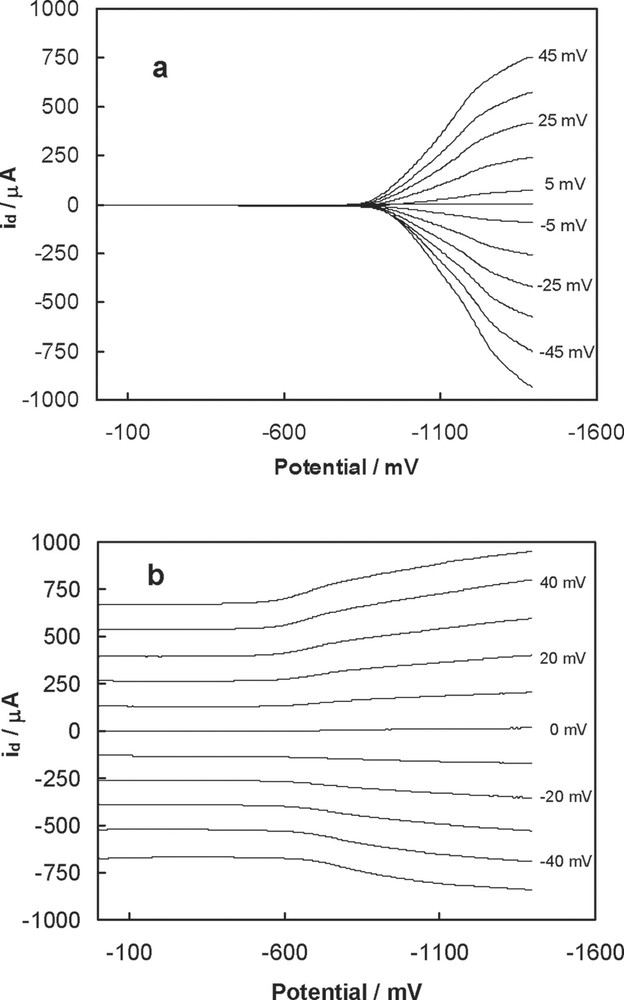
Dependences of the id current (see Fig. 8a) on the potential for C60/Pd films electropolymerized from Pd(CH3CO2)2 and C60 with platinum interdigitated microelectrode arrays in acetonitrile containing 0.10 M tetra(n-butyl)ammonium perchlorate. The C60/Pd films were grown under cyclic voltammetry conditions in acetonitrile/toluene (1:4, v/v) containing 0.10 M tetra(n-butyl)ammonium perchlorate, 0.27 mM C60, and (a) 0.45 mM [Pd(CH3CO2)2]3 or (b) 10.2 mM [Pd(CH3CO2)2]3. K. Winkler, unpublished data.
5 Electropolymerization of transition-metal complexes and fullerene derivatives
Polymers of transition-metal complexes and C60 described above exhibit only n-doping. These polymeric chains undergo reduction and the transport of cations of the supporting electrolyte in and out of the film. Polymers containing fullerene moieties that can undergo p- and n-doping can also be prepared electrochemically. These ‘double cables’ can be divided into two classes:
- ● fullerenes can be incorporated into an electron-donating conjugated polymeric backbone [32–35],
- ● the electron donating groups can be bonded to the polymeric chain of fullerenes [43,44,55].
Polymers which are formed by electroreduction in solution containing Pd(II) or Pt(II) complexes and fullerene derivatives containing electron-donating groups belong to the second class of polymers. Fullerene C60 with covalently linked zinc meso-tetraphenylporphyrin (1), ZnPo–C60 or ZnPp–C60, and 2′-ferrocenylpyrrolidino[3′,4′;1,2]C60fullerene (2) were used as a precursors for electropolymerization. Figs. 14 and 15 show the electrochemical properties of monomers (1) and (2), voltammograms of film formation and electrochemical properties of formed polymers of (1), Fc–C60/Pd, and (2), Znp–C60/Pd, in acetonitrile containing supporting electrolyte. Films are formed under similar conditions as described previously for C60/Pd films. Upon repeated scanning of the potential between 0 and –1200 mV, an increase of the current in the potential range of reduction of fullerene moieties is observed. This behavior indicates the formation of an electrochemically active deposit on the electrode surface. The morphology of Fc–C60/Pd, and Znp–C60/Pd is shown in Fig. 16. It is similar to the morphology of C60/Pd film.
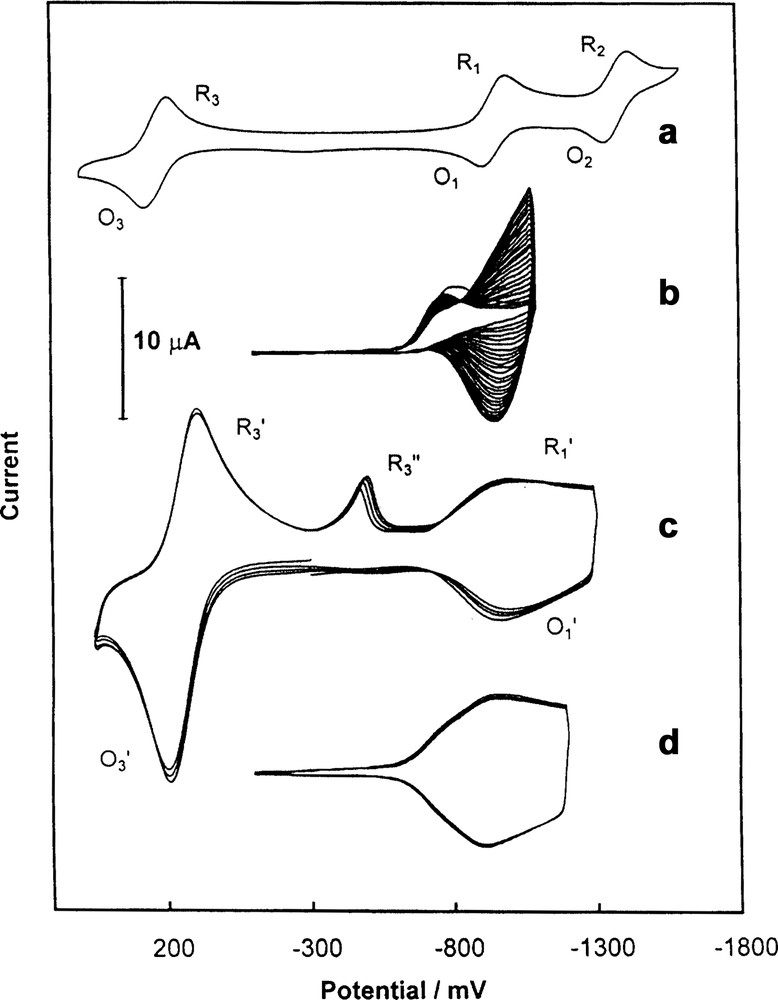
(a) Cyclic voltammogram obtained for 0.31 mM Fc–C60 (2) in acetonitrile/toluene (1:4, v/v) containing 0.10 M tetra(n-butyl)ammonium perchlorate at Au (1.5-mm diameter) electrode. (b) Multicyclic voltammogram obtained for 0.31 mM Fc-C60 (2) and 0.55 mM Pd(CH3CO2)2 in acetonitrile/toluene (1:4, v/v) containing 0.10 M tetra(n-butyl)ammonium perchlorate at Au (1.5 mm diameter) electrode. (c) Multicyclic voltammogram of Au (1.5 mm diameter) electrode covered with Fc–C60/Pd in acetonitrile containing 0.10 M tetra(n-butyl)ammonium perchlorate. The sweep rate was 100 mV/s [43].
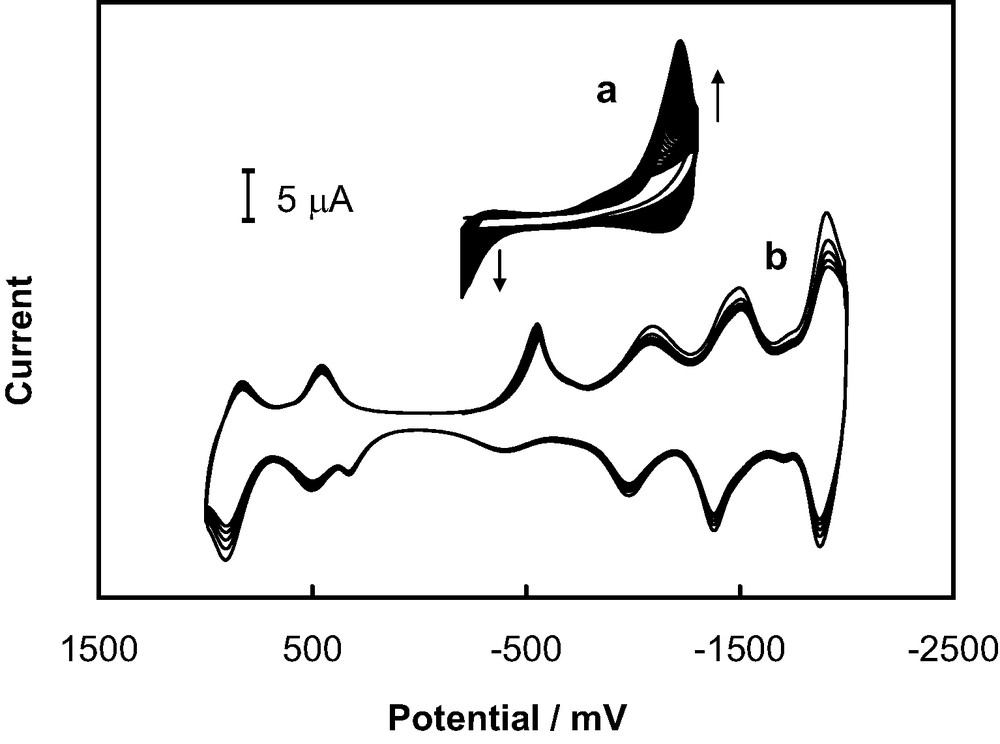
(a) (a) Multicyclic voltammogram obtained for 0.20 mM ZnPp-C60 (1) and 3.12 mM Pd(CH3CO2)2 in acetonitrile/toluene (1/4, v/v) containing 0.10 M tetra(n-butyl)ammonium perchlorate at Au (1.5-mm diameter) electrode. (b) Multicyclic voltammogram of Au (1.5-mm diameter) electrode covered with ZnPp–C60/Pd in acetonitrile containing 0.10 M tetra(n-butyl)ammonium perchlorate. The sweep rate was 100 mV/s [55].
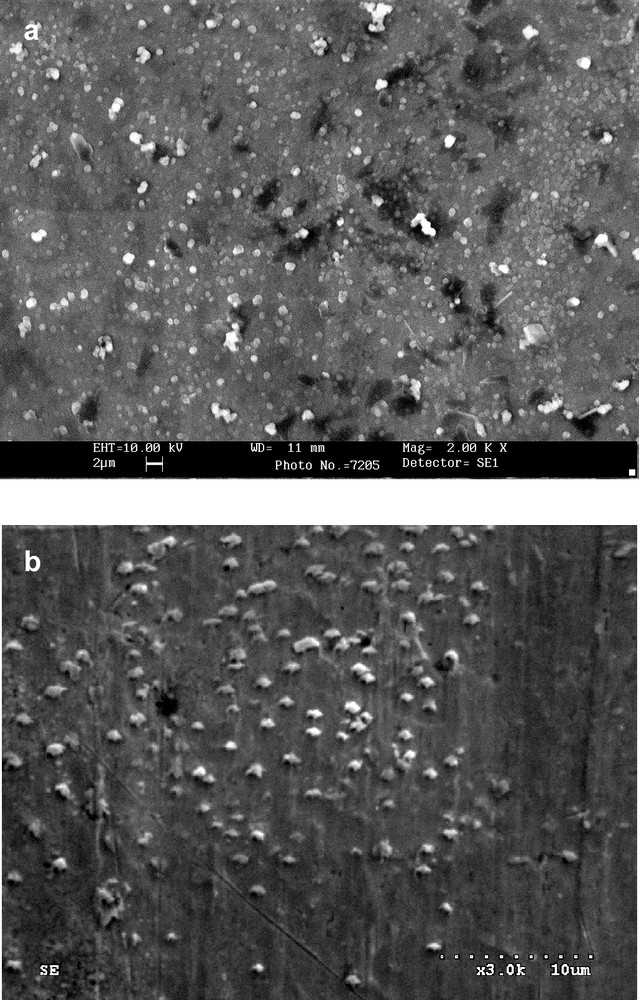
Scanning electron microscopic images of electropolymerized films from (a) Pd(CH3CO2)2/Fc–C60 (2) and (b) Pd(CH3CO2)2/ZnPp–C60 (1). Films were deposited at platinum foil under cyclic voltammetry conditions [43,55].
Films of Fc–C60/Pd, and ZnP–C60/Pd exhibit redox activity both in negative and positive potential ranges. The broad peaks observed in acetonitrile containing tetra(n-butyl)ammonium perchlorate at negative potentials are related to the fullerene electrode processes. For the Fc–C60/Pd film, this process can be described by the following equation:
The reduction of fullerene moieties is accompanied by the transport of cations from the supporting electrolyte into the solid phase. The voltammetric behavior of the film in negative potential range resembles the properties of typical conducting polymers such as polypyrrole [56,57], polyaniline [58,59] or polythiophene [60–62], which, however, are redox-active only at positive potentials.
The sharp and symmetrical peaks that appear at positive potentials are related to the process of ferrocene group oxidation according to the reaction:
The electron transfer occurs via a process of sequential electron self-exchange between neighboring ferrocene groups. The charge percolation through the layer is accompanied by the transport of anions from the supporting electrolyte. In the positive potential range, the Fc–C60/Pd film exhibits voltammetric behavior typical for redox polymers [63].
Similar behavior was observed for Fc–C60/Pt film [44]. In the case of ZnP–C60/Pd, two oxidation peaks related to the oxidation of zinc porphyrin are observed [55].
The presence of large groups on the fullerene surface influences the process of polymer formation. For the Fc–C60/Pd [43] and the ZnP–C60/Pd [55] films, the yield of film formation is lower than that obtained for pristine C60. A very low yield of film was obtained during electroreduction of an acetonitrile solution containing Fc–C60 (2) and Ir(CO)2Cl(p-toluidine) [44]. The very thin film formed in this case is not electrochemically active. For a solution containing Fc–C60 (2) and Rh2(CF3CO2)4, no polymer is formed under electrochemical conditions [44].
6 Applications of the films formed by reduction of fullerenes and transition-metal complexes
Electrochemically formed films of fullerenes or their derivatives and transition-metal complexes have a considerable number of potential applications. They can be used as charge storage materials for batteries and photovoltaic devices. For these applications, the electroactive material should exhibit excellent reversibility, long cycle life and high capacitance [64].
In the case of C60/Pd or C60/Pt films, two kinds of capacitors can be considered:
- ● a pseudocapacitor formed in solution with relatively low ratio of the concentration of palladium or platinum complex to the concentration of C60 in the growth solution,
- ● a double layer capacitor electropolymerized from a solution containing a large excess of palladium complex in the growth solution. In this case the resulting film will contain a significant amount of palladium or platinum nanoparticles.
The C60/Pd pseudocapacitors exhibit conductivity in the potential range needed for film reduction. In this case, the faradaic process of C60 reduction gives rise to the pseudocapacitance. Fig. 17 shows cyclic voltammograms that demonstrate the capacitive behavior of C60/Pd films in acetonitrile solutions containing different tetra(alkyl)ammonium perchlorates as supporting electrolytes. These voltammograms show pseudorectangular cathodic and anodic profiles that show the mirror image relationship that is characteristic of an ideal capacitor. The current depends linearly on a sweep rate. The film can be cycled between –750 and –1500 mV without noticeable change of the shape of voltammograms. The specific pseudocapacitance depends on the size of the cation in the supporting electrolyte. Values of the specific pseudocapacitance obtained with different tetra(alkyl)ammonium cations are collected in Table 2.
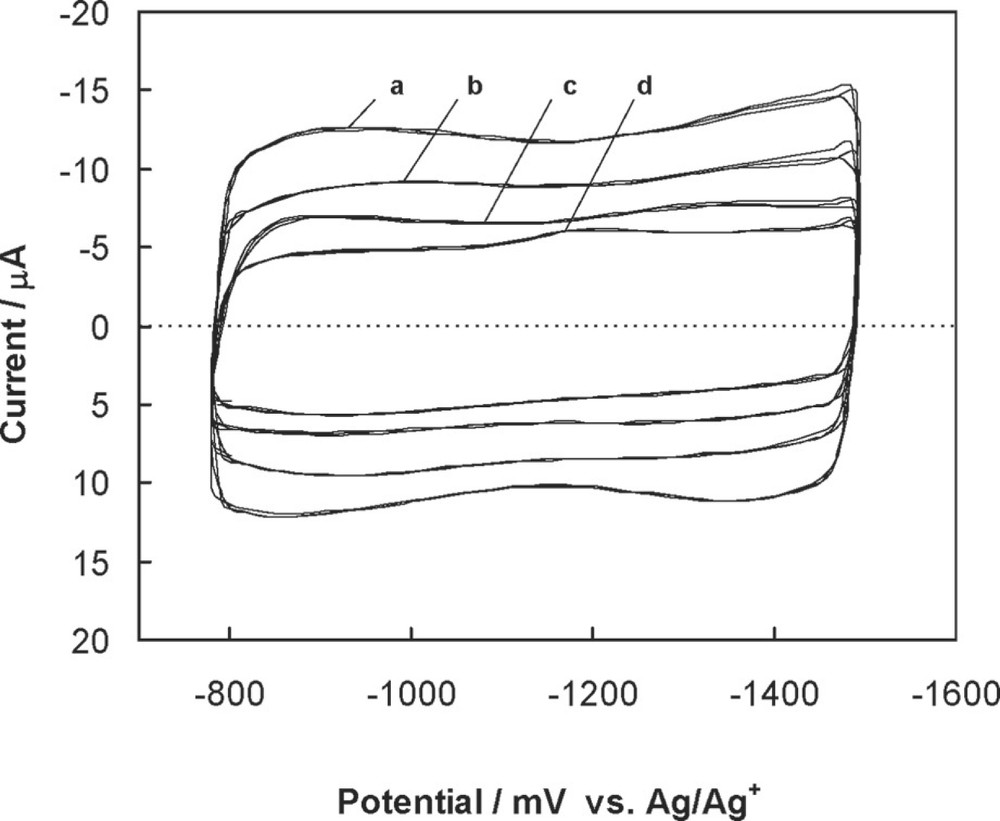
Cyclic voltammograms showing the capacitive behavior of films electropolymerized from Pd(CH3CO2)2 and C60 in acetonitrile containing: (a) 0.06 M tetra(methyl)ammonium perchlorate, (b) 0.10 M tetra(ethyl)ammonium perchlorate, (c) 0.10 M tetra(n-butyl)ammonium perchlorate, and (d) 0.10 M tetra(n-hexyl)ammonium perchlorate. Sweep rate was 50 mV/s. K. Winkler, unpublished data.
Specific capacity of C60/Pd films in acetonitrile containing tetra(alkyl)ammonium perchlorates
Supporting electrolyte | Specific capacity | (F/g) |
(n-Hx)4NClO4 | 160 a | 49 b |
(n-Bu)4NClO4 | 195 a | 53 b |
(Et)4NClO4 | 255 a | 57 b |
(Me)4NClO4 | 310 a |
a Film formed in acetonitrile/toluene (1:4, v/v) containing 0.10 M tetra(n-butyl)ammonium perchlorate, 0.27 mM C60 and 3.6 mM Pd(CH3CO2)2. The specific capacitance was calculated for voltammograms recorded in the potential range from –800 to –1500 mV.
b Film rich in Pd nanoclusters formed in acetonitrile/toluene (1:4, v/v) containing 0.10 M tetra(n-butyl)ammonium perchlorate, 0.27 mM C60 and 8.9 mM Pd(CH3CO2)2. The specific capacitance was calculated for voltammograms recorded in the potential range from 0 to –700 mV.
The C60/Pd films containing large quantities of palladium nanoparticles exhibit behavior that is characteristic of double layer capacitors. These films are conductive over the large potential range from +200 to –1800 mV. The specific capacitance of these films is lower than the specific capacitance of films formed in solution with low ratio of concentrations of palladium complex to C60, which lack the metallic nanoparticles A value of 50 F/g was obtained for the C60/Pd double layer capacitor in acetonitrile containing tetra(n-butyl)ammonium perchlorate. This value is in the range of specific capacitance of typical double layer capacitors [65].
The C60/Pd film can be also used as an electrochemical sensor. For example, the electrochemical behavior of the C60/Pd film is altered when either carbon monoxide or imidazole is added. As seen in Fig. 18, the electrochemical response of an electrode covered with the C60/Pd film becomes more reversible in the presence of carbon monoxide, and the reduction potential of the treated film shifts toward less negative values. The effect of imidazole on the voltammetric behavior of C60/Pd film is less pronounced. But again the presence of imidazole in solution results in a shift of the reduction potential of the C60/Pd film toward less negative values. Presumably these changes in the redox response of the film results in part from coordination of carbon monoxide or imidazole to the palladium atoms within the polymer. C60/Pd films were also used as a catalyst for cytochrome c electroreduction [66]. In this case, the incorporation of cytochrome c into the structure of the polymeric film is responsible for observed catalytic effect.
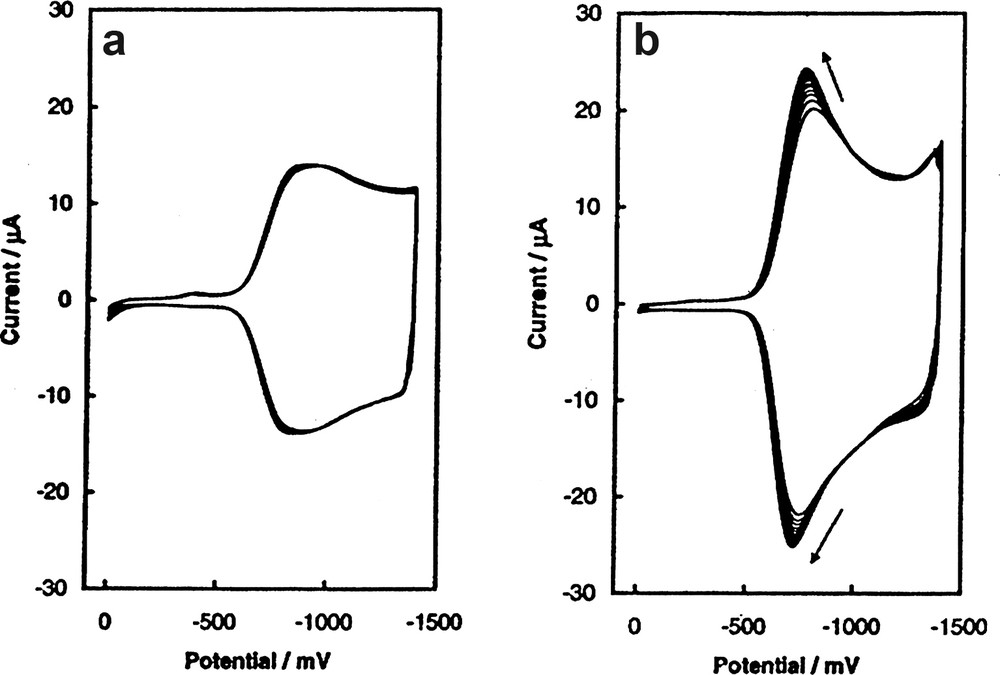
Cyclic voltammograms of the C60/Pd film electropolymerized from Pd(CH3CO2)2 and C60 in acetonitrile containing 0.10 M tetra(ethyl)ammonium perchlorate (a) before, and (b) after treatment with carbon monoxide. The sweep rate was 100 mV/s. K. Winkler, A.L. Balch, unpublished data.
Recently, films of palladium and fulleropyrrolidine bearing covalently attached crown ethers have been synthesized under electrochemical conditions [67]. These films can be used as sensors for alkali metal cations. The crown ether portion can selectively bond the alkali metal cations depending on the size of crown ether cavity. Fig. 19 shows the changes of the potential of an electrode modified with the (18-C-6)–C60/Pd film after addition of solutions containing either K+ or Cs+ ions.
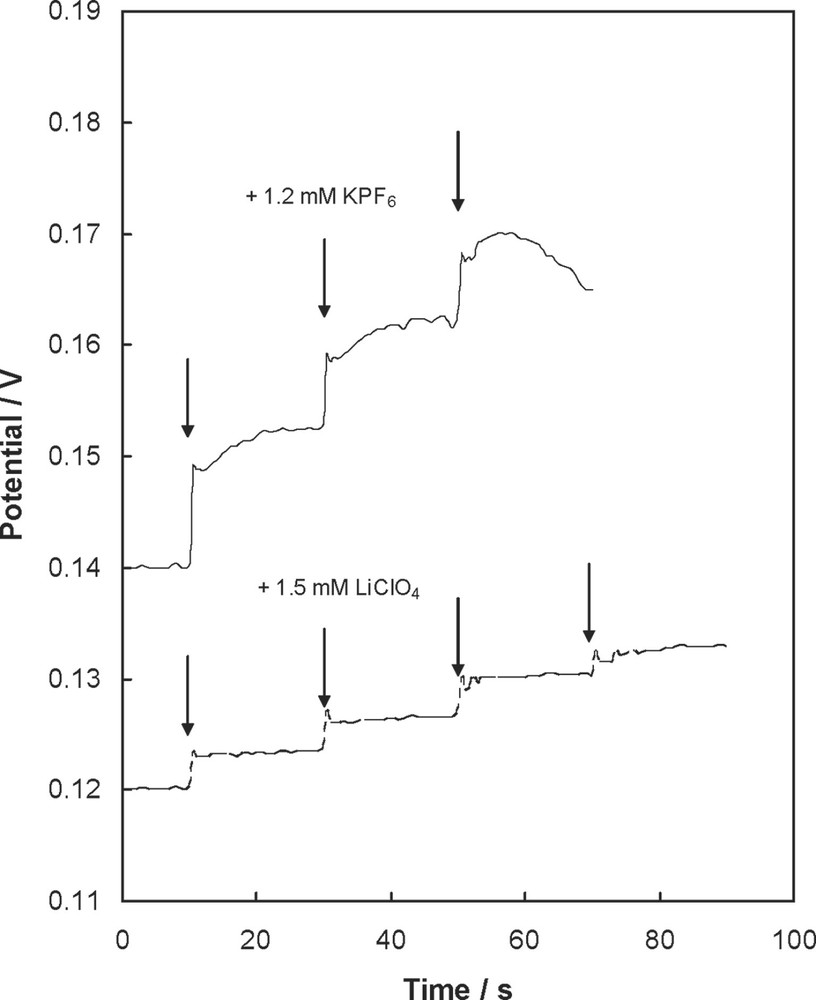
The changes of potential of a gold electrode modified with the (18-C-6)–C60/Pd film immersed in acetonitrile containing 0.10 M tetra(n-butyl)ammonium tetrafluoroborate during addition of solutions containing either LiClO4 or KPF6 (K. Winkler, F. D'Sousa, A.L. Balch, unpublished data).
The C60/Pd film was also used for the preparation of polymer bilayers [68]. A bilayer composed of an inner layer of polypyrrole and an outer layer of C60/Pd is redox-active over an unusually broad potential range (Fig. 20). The inner polypyrrole layer exhibits p-doped properties, while the C60/Pd outer layer undergoes n-doping. The charge transfer between these two layers is possible because the energies of the conducting levels of the two individual components of the film overlap. The porous structure of the inner C60/Pd layer allows transport of supporting electrolyte ions into the inner layer during its oxidation. The electrochemical properties of this layer depend on the solvent and the supporting electrolyte.
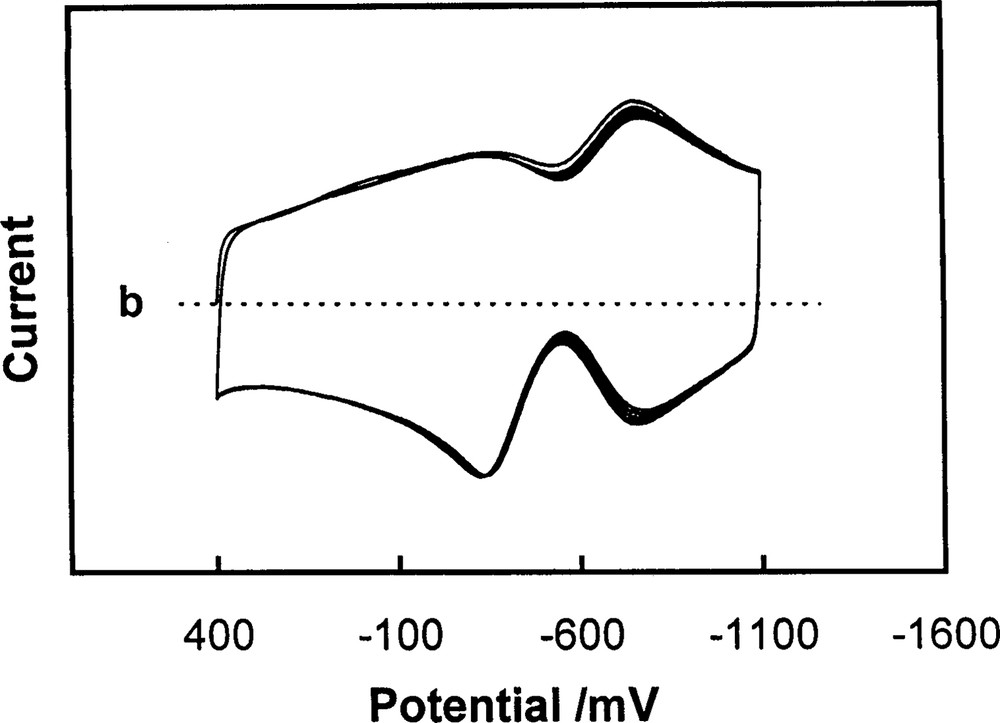
Multicyclic voltammogram of an electrode/polypyrrole/C60Pd bilayer in acetonitrile containing 0.10 M tetra(ethyl)ammonium perchlorate. The sweep rate was 100 mV/s [68].
Chemically produced C60/Pd polymers have been used as catalysts for hydrogenation of olefins and acetylenes [53,69]. The catalytic activity of this system depends on the ratio of C60 to palladium or platinum in the polymer. There are two kinds of metal atoms in the polymer. The palladium or platinum atoms that connect the fullerene cages apparently do not exhibit catalytic activity. Rather, the hydrogenation reactions are catalyzed by palladium or platinum nanoparticles present on the surface of the polymer [69]. Similar catalytic properties can be expected for electrochemically formed films containing large amounts of palladium or platinum nanoparticles.
Chemically produced C60/Pd has been used a gas adsorbent [70]. It was shown that toluene is adsorbed within this polymer. Again, the amount of toluene adsorbed by the C60/Pd polymer depends on its composition. It has been suggested that interaction of the π-electrons of toluene with Pd atoms involved in formation of the polymeric chain is responsible for adsorption phenomena. Similar behavior was observed for the C60/Pt polymer [70]. However, chemically prepared C60/Ru(CO)n does not adsorb toluene. In this case, CO ligands from the starting material bound to the ruthenium prevent the adsorption of toluene by the polymer.
Laser ablation of electrochemically formed C60/M and C70/M films (M = Pt or Ir(CO)2) results in fragmentation and formation of heterofullerenes such as C59M+ and C58M– [71,72]. The structures of these novel hetero-fullerenes with metal centers incorporated within the carbon framework have been computed using density functional techniques [71–73]. Fig. 21 shows the computed structures of these heterofullerenes with the metal atoms substituting for one or two carbon atoms within the fullerene cage. These results demonstrate the possible application of the C60/M and C70/M polymers for preparation of new hetero-fullerenes.
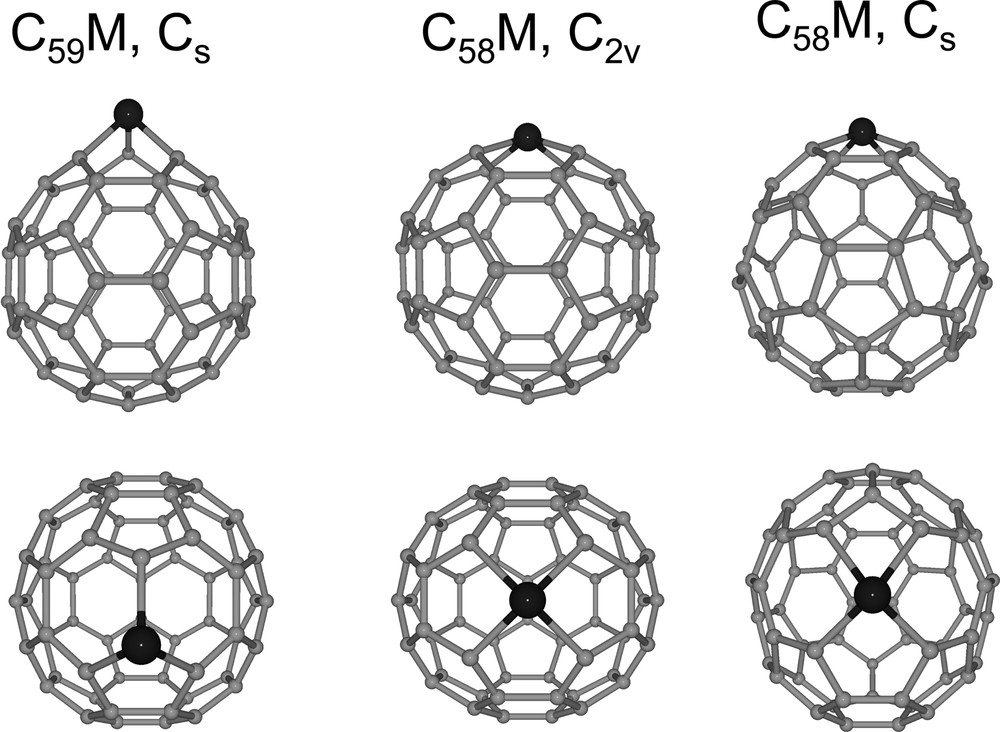
Two orthogonal views of the calculated structures of C59M, the C2v isomer of C58M (6:6 C–C bond substitution), and the Cs isomer of C58M (6:5 substitution) [71,72].
7 Conclusion
This article has reviewed the preparation and properties of redox-active films formed by the electrochemical reduction of fullerenes and selected transition-metal complexes. These films adhere to the electrode surface and retain their electrochemical activity when transferred to solutions containing only supporting electrolyte. They may be prepared with a variety of metal complexes and with chemically modified fullerenes. The electrochemical properties of these films have been investigated and the effects of incorporation of metallic nanoparticles within the films have been examined. The films show high pseudocapacitance and reversible charging and discharging. Preliminary studies have show they can be utilized as sensors, catalysts, components in bilayer construction, gas adsorbents, and as synthetic precursors to metal complexes and heterofullerenes. The number of uses of these films can be expected to grow as their properties are recognized.
Acknowledgments
Financial support of the State Committee for Scientific Research, Poland (project 3T09A04626 to K.W.) and the National Science Foundation (grant CHE 0413857 to A.L.B.) is gratefully acknowledged.