1 Introduction
Carbohydrates located at the surface of cells are involved in most of the major biological events. They are found in the form of glycolipids or glycoproteins at the outer layer of the cell membrane, where they serve as biological markers.
Glycolipids are found in prokaryotic cells, e.g. LPS of Gram(−) bacteriae, lipoteichoic acids of Gram (+) bacteriae, glycoglycerolipids of archaebacteriae, or trehalose-containing lipopolysaccharides and phenolic glycolipids of mycobacteriae. In plants, they are mostly found as glycosyl diacylglycerols or steryl glycolipids. In animals, they are found as glycosyl ceramides (cerebrosides, globosides, gangliosides) at the surface of the plasma membrane of tissues or at the surface of red blood cells [1].
Glycoproteins are also found in the bacterial, vegetal and animal kingdoms as well; they can be inserted into the bilayer membrane either through embedment or by way of a GPI anchor. Their carbohydrate content can vary from less than 1% of the total weight of the glycoprotein (in some plant lectins) to more than 80% (in blood group glycoproteins) [2,3].
Important biological roles of the surface glycans lie in their property to serve as ligand in various recognition phenomena involving protein receptors. Blood group substances/immunoglobulins, bacterial glycan antigens/antibodies or carbohydrate ligands/selectins are some examples [4]. This role can be well understood taking into account the enormous potential of oligosaccharides in the encoding of biological informations [5]. The recognition phenomena are involved in major biological events such as cell adhesion, cell growth and proliferation, immunological defence against invading hosts, integrity or apoptose of cells, fertilization and so on [4].
Since they serve as biological markers, carbohydrates can be used as haptens for immunological purposes (diagnosis, artificial antigens [6]), as cell pathogenicity markers or as adhesion molecules [7].
For that purpose, they must be conjugated with a carrier that can be a polymer, a protein or a lipid in order to present them to the biological environment in a high molecular weight and highly organized assembly [8]. The carbohydrate–protein recognition phenomenon can be detected and quantified in several manners in order to develop diagnosis or biosensors devices (e.g. precipitin reactions, fluorescence, use of tracers, ELISA technique). This short review focuses on a simple technique, based on color changes in the UV–visible spectrum.
1.1 Polydiacetylenic polymers and color changes
Conjugated diacetylenic acids (Scheme 1) were shown since 1972 to polymerize by UV irradiation to red colored conjugated enynes systems [9], either in the solid state or in oriented mono- or multilayers. Later, diacetylenic phospholipidic vesicles were polymerized to stable vesicles that can change their color from colorless, to blue then red during polymerization or directly from colorless to red, depending on their structure [10].

Conjugated diynoic acids most often used in the formation of color changeable vesicles.
The photochemical polymerization required a topochemical organization of the diacetylenic monomers and it was studied in details as well in monolayers (or multilayers) as in vesicles. Optical absorption in polydiacetylene occurs via a π-to-π∗ absorption within the linear π-conjugated polymer backbone. The conjugated polydiacetylene backbone has two spectroscopically distinct phases, designated as the blue and the red forms, that result from their excitation absorption peaks at 650 and 540 nm, respectively. Under external perturbation, such as heat or mechanical stress, the conjugated polydiacetylene backbone can undergo a color change from the blue to the red form. The mechanism of the color change is not understood in detail. It is believed that molecular conformation changes, such as side chain packing, ordering, and orientation, impart a stress to the polymer backbone that alters its conformation, thus changing the electronic state and the corresponding optical absorption [11–21]. Synthetic glycolipids bearing a conjugated diacetylenic structure were also copolymerized with 10,12-diynoic acids. Their carbohydrate moieties varied from monosaccharide (1–6) to di or trisaccharide units (7–9); their polymerizable alkyl chain were pentacosa-10,12-diynoic acid (4–9) or hexacosa-10,12-diynoic acid (2–3). Most often, a linker was placed between the hydrophilic and hydrophobic moieties. Several of them are reported in Table 1.
Synthetic 10,12-diyne glycolipids successfully copolymerized with 10,12-diynoic acid in topochemical organizations
Compound | Ref. | Carbohydrate head | Polymerisable diyne |
1 | [29] | ||
2 | [29] | ||
3 | [29,30] | ||
4 | [31] | ||
5 | [32,33] | ||
6 | [13,22–27] | ||
7 | [22,25,27,28] | ||
8 | [28] | ||
9 | [27,28] |
It was shown most often that the polymerizable glycolipids do not avoid the polymerization of α,γ-diynoic monomers, as far as the topochemical organization was retained. The copolymers thus obtained were endowed with the same color change properties as the polymers of pure diynoic monomers. It was also demonstrated that the non-covalent embedment of natural ceramides such as GM1 (10) [21,25,26,34–36] or GT1b (11) [25] (Scheme 2) in organized assemblies (monolayers, vesicles) of pentacosa-10,12-diynoic acid do not prevent the polymerization of the latter, if the topochemical organization is not disrupted.
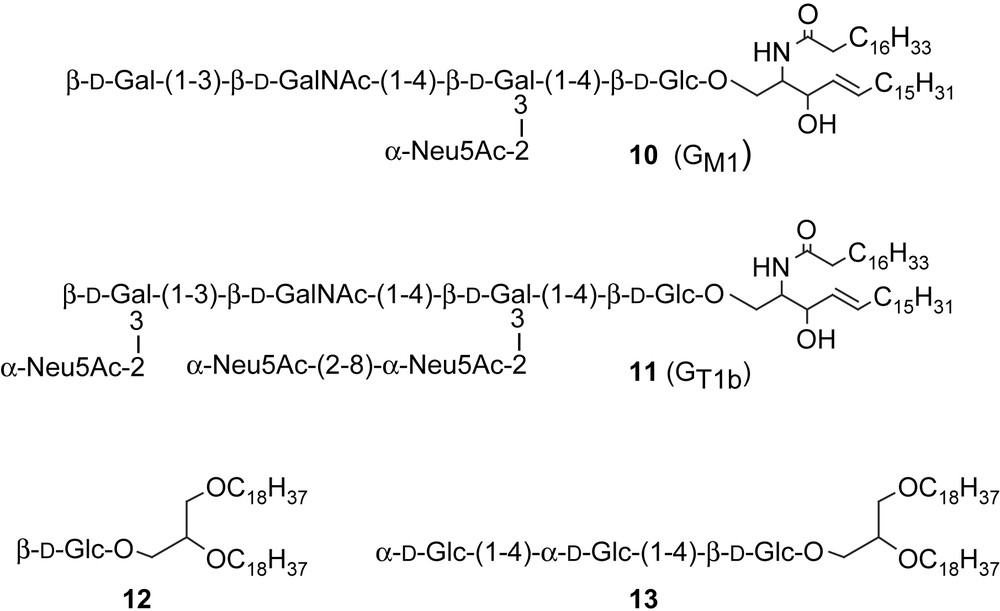
Natural and synthetic glycolipids embedded into polydiacetylenic vesicles.
1.2 Color change induced by carbohydrate recognition
The most interesting feature, with regards to molecular recognition, was reported by Charych et al. who demonstrated that the color change could be induced by the recognition of a carbohydrate embedded into a polydiacetylene monolayer. Thus, the polymerization of a monolayer composed of pentacosa-10,12-diynoic acid and a N-acetyl neuraminic acid neoglycolipid bearing a diacetylene hydrophobic moiety (6) gave rise to a blue monolayer that turned to red when exposed to influenza virus [22]. Since then, several glycolipids embedded into polymerized diacetylenic backbones were used as ligands for molecular recognition towards microorganisms or proteins. Thus compound 6 could cause a color change in the presence of influenza virus [22,25,27], whereas the lactose counterpart 7 did not [22–25]; the sialyl Lewisx analogue 9 was recognized by a P-selectin [28], GM1 and GT1b gangliosides displayed selectivity against cholera neurotoxin and botulinum neurotoxin, respectively [25,34], GM1 binding to E. coli enterotoxin was quantified by amperometric measurement on redox vesicles [35,36], mannose glycolipid (5) was used for the detection of E. coli either by colorimetric [32] or electrochemical measurement [33].
The colorimetric response (CR) was usually quantified as:
CR = [B0 − B]/[B0] × 100%, with B = Ab/(Ab + Ar) |
This unique chromatic property has made polydiacetylene a promising candidate in the development of biosensors [22,24,27,32–34,38–42]. Polydiacetylene vesicles or Langmuir–Blodgett (LB) films covered with virus-specific or toxin-specific ligands undergo dramatic color changes from blue to red in direct response to pathogen binding at the interface. These biochromic effects arise from (1) multipoint interactions of the receptor with the polydiacetylene backbone, and/or (2) insertion of viral membrane or toxin hydrophobic domains into the polydiacetylene network [27]. A major drawback to such development is the availability of the polymerizable glycolipids, since conjugated diacetylenic compounds are not easy to prepare [43] and the synthesis of oligosaccharides is time consuming.
It was already reported that natural glycolipids 10 and 11 could be inserted into the polydiacetylene matrix of pentacosa-10,12-diynoic acid (10,12-PCDA) based on non-covalent binding [21,25,26,34–36]. Also, we decided to study the potential of simpler synthetic neoglycolipids embedded into polymerized vesicles in order to optimize the conditions in which they could serve as ligands for molecular recognition towards proteins and cells.
2 Discussion
2.1 Synthesis of neoglycolipids
In order to serve as ligand and induce a color change, the carbohydrate should be located at a distance from the surface of the polymer matrix that is neither too close (in order not to hinder the approach of the complementary protein or microorganism), nor too far (in order to induce a stress at the interface during recognition). Our previous results in the field of molecular recognition of glycolipids at the surface of liposomes or mixed phospholipid/glycolipid monolayers [44–46] induced us to prepare compounds in which the carbohydrate head is separated from the hydrophobic anchor by hydrophilic spacers of various lengths (Scheme 3).
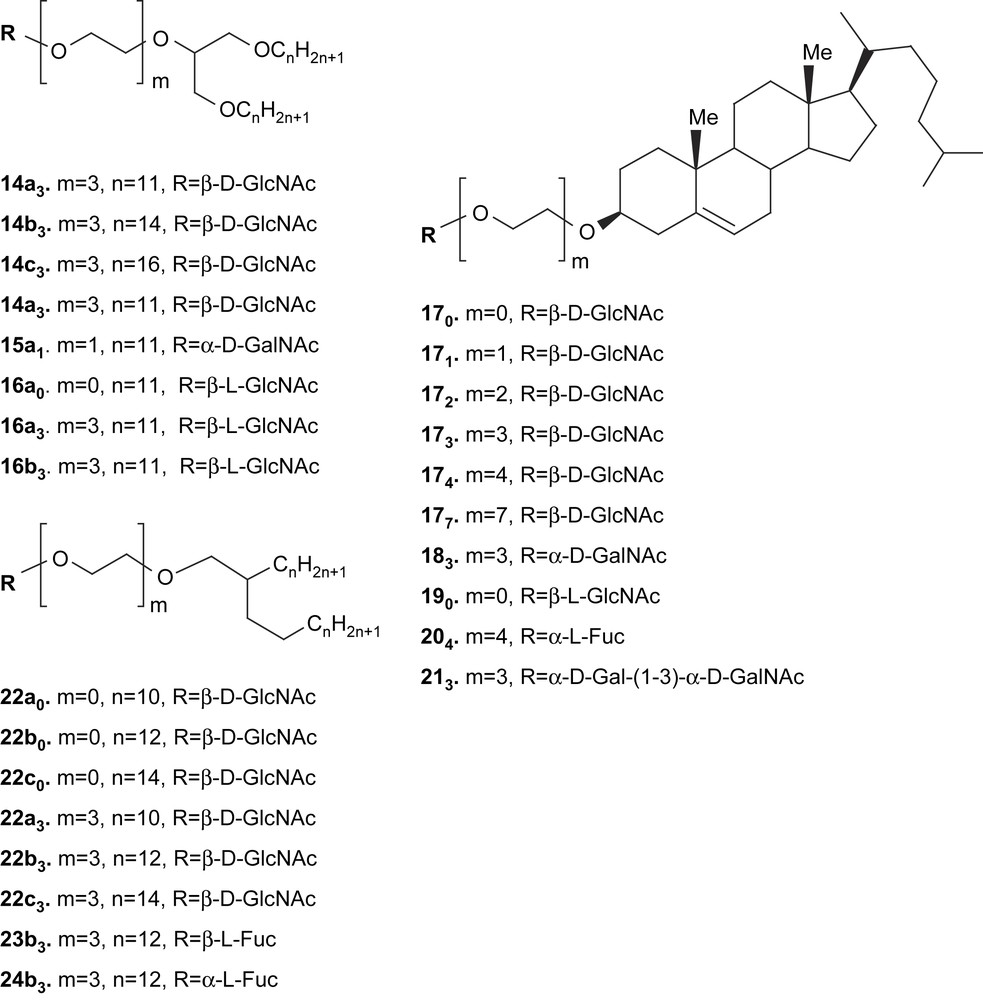
Synthetic glycolipids prepared for embedment into polydiacetylene monolayers and vesicles.
The series of compounds 14–16 were shown to possess remarkable properties with regard to their monolayers assemblies. Such assemblies were stable, transferable and able to retain proteins at the interface [47–49]. As expected, cholesteryl compounds 17–20 were good candidates for the embedment into liposomes. The GlcNAc series of glycolipids (17) was shown to incorporate into phospholipids liposomes up to high ratios and to be recognized by a specific lectin (WGA) at the interface [44,50]. Furthermore, compounds 174 and 204 were shown to have a fluidizing effect on phospholipid bilayers [51]. Glycolipids derivatives of Guerbet's alcohols (22–24) are also endowed with interesting organization properties [44,45,46,52,53]. In liposomes assemblies, the fucosyl derivatives 204, 23b3 and 24b3 were shown to display recognition properties and a high stereospecificity against three different lectins, i.e. wheat germ (WGA), Ulex europeaus I (UEA-I) and Lotus tetragonolobus (LTA) agglutinins [46].
The syntheses of most of these compounds have already been published; only the most important steps will be underlined in the present paper. Two important sets of reactions have to be taken into account: the first one is the synthesis of the hydrophobic moiety (that is often amphiphilic in itself), the second one is the condensation of the carbohydrate moiety. Scheme 4 is a short description of the synthesis of the acceptor alcohols 25m–27m.

Short description of the reaction pathways for the preparation of acceptor amphiphilic alcohols. (a) CnH2n+1OH, NaH, DMF; (b) Cl(CH2CH2O)m−1CH2CH2Cl, NaOH, Bu4NHSO4; (c) HCOONa, DMF, Bu4NBr, then aq. 12 M NaOH; (d) TsO(CH2CH2O)mOTHP, NaH, DMF; (e) aq. 0.1 N HCl; (f) HO(CH2CH2O)mH; (g) ClCH2CH2OCH2CH2Cl, NaOH, Bu4NHSO4, then (c).
Compounds 25m were prepared from epichlorohydrin and an excess of alkyl alcohol in alkaline medium. After purification, the intermediate was reacted in phase-transfer conditions with an oligoethylene glycol dichloride; the terminal unreacted chlorine atom was then hydrolyzed in two steps, e.g. substitution by a formate and then saponification of the latter [47]. One other reaction pathway was followed for the preparation of derivatives 26m, from commercial Guerbet's alcohols: condensation of an oligoethylene glycol protected on one hydroxyl function with a tetrahydropyranyl group and activated on the other one via a tosylate, then acidic hydrolysis of the tetrahydropyranyl ether [54]. It should be mentioned that the same coupling was also realized on glycerol 1,3-diethers. In the case of derivatives 27m, the tosylation was realized on the cholesterol moiety and an excess of oligoethylene glycol was condensed on the latter. For m > 4, monodisperse compounds are not commercially available and they were prepared by stepwise elongation of the oligoethylene glycol unit via condensation of diethyleneglycol dichloride and hydrolysis of the terminal chlorine atom as described previously [50].
After preparation of the alcohols 25m–27m, glycosylations were realized with various donors and promoters whose structures were chosen in terms of nature and stereochemistry of the final expected neoglycolipid. A short description is given in Scheme 5.

General procedure for the preparation of neoglycolipids 14–24 and structures of the glycosylation donors used for such syntheses.
For the preparation of β-d-N-acetylglucosamine derivatives (14, 17, 22), we used donor 28 [55] with a stoichiometric amount of trimethylsilyl triflate (TMSOTf) as the promoter in CH2Cl2 at low temperature. The expected glycosides were obtained in excellent yields and 1,2-trans-stereoselectivity. The method was recently extended to the preparation of β-l-N-acetylglucosamine enantiomers 16 by changing the anomeric leaving group to a thioethyl or a pentenyl function (29); also iodoazide 30 was used for the preparation of 190 [56]. The preparation of α-d-N-acetylgalactosamine derivatives 15a1 and 183 required a donor able to induce 1,2-cis-stereochemistry. Compound 31 [57] afforded the highest yields and best stereoselectivity to the expected glycosides, using catalytic amounts of TMSOTf as the promoter and diethyl ether as the solvent [58]. For the preparation of l-fucopyranosides 204, 23b3 and 24b3, two different donors were used, depending on the expected final stereochemistry. Donor 32 [59] was found to give the best yields and 1,2-cis-stereoselectivity to 204 and 24b3 in comparison with other donors, when Bu4NBr was used as the promoter, whereas donor 33 [60] was the best one to induce the 1,2-trans-stereoselectivity towards compound 23b3, in the presence of silver carbonate as the promoter [53]. Finally, the fully protected glycolipids thus obtained were deprotected in the usual manners: O-acetyl groups were removed by Zemplén de-O-acetylation, the N-allyloxycarbonyl groups were cleaved with Pd(PPh3)4 in the presence of dimethyl malonate before re-N-acetylation, the azido groups were hydrogenolyzed to amino derivatives before re-N-acetylation and the benzyl ethers were removed by transfer hydrogenation (cyclohexene, Pd(OH)2). All the compounds depicted in Scheme 3 were prepared in good yields and fully characterized. Several of them were used as target for cells or proteins at the surface of polymerized diacetylenic vesicles, several others are under investigation.
2.2 Color response to E. coli with regard to the nature of the polydiacetylenic/glycolipid assembly
Vesicles of tricosa-2,4-diynoic acid (2,4-TCDA) or pentacosa-10,12-diynoic acid (10,12-PCDA) as the matrix and a 0.02 molar ratio of a β-glucoside of glycerol dioctadecylether (12, Scheme 2 [61]) were obtained by probe sonication method. When polymerized at 254 nm, the vesicles appeared deeply blue. When exposed to Escherichia coli (E. coli), the vesicles made from 2,4-TCDA turned to red within a few seconds, whereas those made from 10,12-PCDA did not [62,63]. In the case of 2,4-TCDA/12 vesicles, the absorption maximum was shifted from ≈650 nm to ≈540 nm, as recorded in Fig. 1.

Colorimetric detection of E. coli by polymerized diacetylene vesicles containing 2% of glycolipid 12. Visible absorption of vesicle solution; (a) without E. coli, (b) in the presence of E. coli [62].
An explanation of this phenomenon was given by the observation of monolayers of pure 2,4-TCDA or 10,12-PCDA and their mixtures with glycolipid 12.
As can be seen from Fig. 2, the negative deviations from the additivity rule observed for all molecular fractions at all surface pressures indicate that there exist strong interactions and good miscibility between 2,4-TCDA and 12, in water (A) and 10−4 M CdCl2 (B) as well. In contrast, with 10,12-PCDA/12 monolayers, positive deviations from the additivity rule are observed for all molecular fractions at all surface pressures in water (A), thus indicating repulsion between 10,12-PCDA and 12. It could be noted that the repulsion is almost abolished in 10−4 M CdCl2 (B).

Molecular area of the mixed 2,4-TCDA/12 and mixed 10,12-PCDA/12 monolayers as a function of the molar fraction of 12 at different surface pressures: (A) on pure water, pH 5.8; (B) on 10−4 M CdCl2, pH 6.6. Pressure (mN/m): (a) 5.00; (b) 10.00; (c) 20.00; (d) 30.00; (e) 40.00; (f) 15.00 [63].
The interaction was analyzed more quantitatively by measuring the excess free energy of mixing (ΔGxsπ). As can be seen from Fig. 3, ΔGxsπ was negative for all molecular fractions at all surface pressures for 2,4-TCDA/12 monolayers, thus indicating a strong interaction between 2,4-TCDA and 12, as well in water (A) as in 10−4 M CdCl2 (B). Contrarywise, a repulsive effect was observed between 10,12-PCDA and 12 for all molar fractions at all surface pressures in water (A), whereas the mixing effects in 10−4 M CdCl2 (B) were shown to be strongly dependent on the surface pressure and the ratio 12/10,12-PCDA. These results were also confirmed by Brewster angle microscopy (BAM) of the monolayers that show distinct domains near 40 mN/m, indicating phase separation in the monolayer 12/10,12-PCDA, whereas no domains were visible at the same pressure for monolayers of 12/2,4-TCDA.

Excess free energies of mixing as a function of the molar fraction of 12 for mixed 2,4-TCDA/12 and mixed 10,12-PCDA/12 monolayers at different surface pressures: (A) on pure water, pH 5.8; (B) on 10−4 M CdCl2, pH 6.6. Pressure (mN/m): (a) 5.00; (b) 10.00; (c) 20.00; (d) 30.00; (e) 15.00 [63].
It seemed therefore that the color changes induced by molecular recognition are strongly dependent on the glycolipid/polydiacetylene array. Further insights were sought by varying the length of the diynoic acid and that of the ethylene glycol spacer in the glycolipid.
2,4-Heneicosadiynoic acid (2,4-HCDA) or 2,4-tricosadiynoic acid (2,4-TCDA) vesicles containing 2% molar ratios of cholesteryl glycolipids 171, 173 or 174 were obtained by sonication of a water suspension. After polymerization by UV irradiation at 254 nm, blue colored solutions were obtained, in each case. When E. coli (dispersed in 0.85% aq NaCl) was added to the latter, they change their color to red within a few seconds, whereas no color change was observed during addition of aq NaCl only, or when E. coli was added to a suspension of vesicles made of 2,4-diynoic acid only.
As displayed in Fig. 4A, for 2,4-HCDA vesicles, the color response was shown to be strongly dependent on the oligoethylene glycol spacer length; the longer the spacer, the higher the color response [64].

(A) Colorimetric response of 2,4-HCDA/17n vesicles incubated with E. coli. □ (n = 1), ● (n = 3), ■ (n = 4). (B) Colorimetric response of vesicles incubated with E. coli. ● (2,4-HCDA/173 vesicles), ■ (2,4-TCDA/173 vesicles) [64].
This result demonstrates that the binding by E. coli is better when the carbohydrate residue is more accessible, but also that the distance between the ligand and the receptor is short enough to induce a stress causing the color change to the polymeric backbone. The role of embedment of the carbohydrate into the polymeric array was further demonstrated by the use of a longer diacetylenic acid. As can be seen in Fig. 4B, the color change in the recognition of glycolipid 173 by E. coli is lower in a 2,4-TCDA polymeric vesicle than in 2,4-HCDA vesicles. It seems reasonable to assume that the glycolipid is more buried into a polymeric backbone made of longer chains and therefore the recognition is lower. The more striking feature is that this effect is observed for chains different by two carbons only [64].
The time needed to observe the color change is very short, as attested by Fig. 5A and 5B. It does not seem to depend to a large extent on the chain lengths or structures of glycolipid. About 70–90% of the color change was observed after 20 s, and nearly completed in 2 min. This very fast phenomenon renders it a good candidate for a biosensor device.

(A) Colorimetric response of 2,4-HCDA/17n vesicles incubated with E. coli. □ (n = 1), ● (n = 3), ■ (n = 4). (B) Colorimetric response of vesicles incubated with E. coli. ● (2,4-HCDA/173 vesicles), ○ (2,4-TCDA/173 vesicles) [64].
2.3 Color response to con A with regard to the burying of the carbohydrate into the polymer backbone and the vesicle sizes
In this study, the recognition was attempted by a molecule of much more smaller size than E. coli, but able to bind N-acetylglucosamine, i.e. the lectin concavalin A (con A). Con A has a good affinity for α-d-glycopyranosides (Man, Glc, GlcNAc), but its lower affinity for β-d-GlcNAc [65] would allow one to detect small differences in recognition that could be hidden with lectins of very high affinity. Concavalin A is a tetramer composed of four identical subunits at pH > 5.6, each containing one carbohydrate, one Mn2+ and one Ca2+ binding sites. Its molecular weight is near 104,000 and its shape roughly globular with a mean diameter of 6 nm [66].
Despite the absence of response of 12/10,12-PCDA vesicles to E. coli [62,63], we decided to use this diacetylenic acid for several reasons: (1) it is more accessible commercially, (2) 10,12-PCDA/glycolipids vesicles were shown to be able to induce color responses in other instances [22–25], (3) the polymeric enyne backbone is moved off the interface, by comparison with 2,4-diynoic compounds, thus allowing a better flexibility at the surface. In order to study the role of embedment in the polymeric network as well as that of the vesicle sizes, we used the series of glycolipids 14a3, 14b3 and 14c3, whose chain lengths varied from 11 to 14 and 16 carbons, respectively.
Glycolipids were mixed with 10,12-PCDA in 5%, 10% or 50% molar ratio. Liposomes were formed by ultrasonication of the lipid suspension in water at 70 °C, then eventually extruded quickly through nylon membranes of various pore size, before polymerization at 254 nm [67]. It was shown that the polymerization of vesicles containing high levels of glycolipids (>50%) was substantially inhibited, probably due to a lack of topochemical orientation of the diacetylene chains.
In a first instance, con A was added to unextruded vesicles made of 10,12-PCDA and glycolipid 14b3 (50% molar ratio). As a result of molecular recognition, the solution turned from blue to red, whereas it has been demonstrated earlier that no color change occurred in the presence of BSA, for example. As shown in Fig. 6, the blue vesicles displayed an UV–visible absorption maximum at 640 nm, and a weaker absorption at 540 nm. In the presence of con A, the maximum at 540 nm increased, whereas that at 640 nm decreased. Though the color change began within a few minutes, 50 min were found to be an average time to reach a plateau in non-stirred solutions.
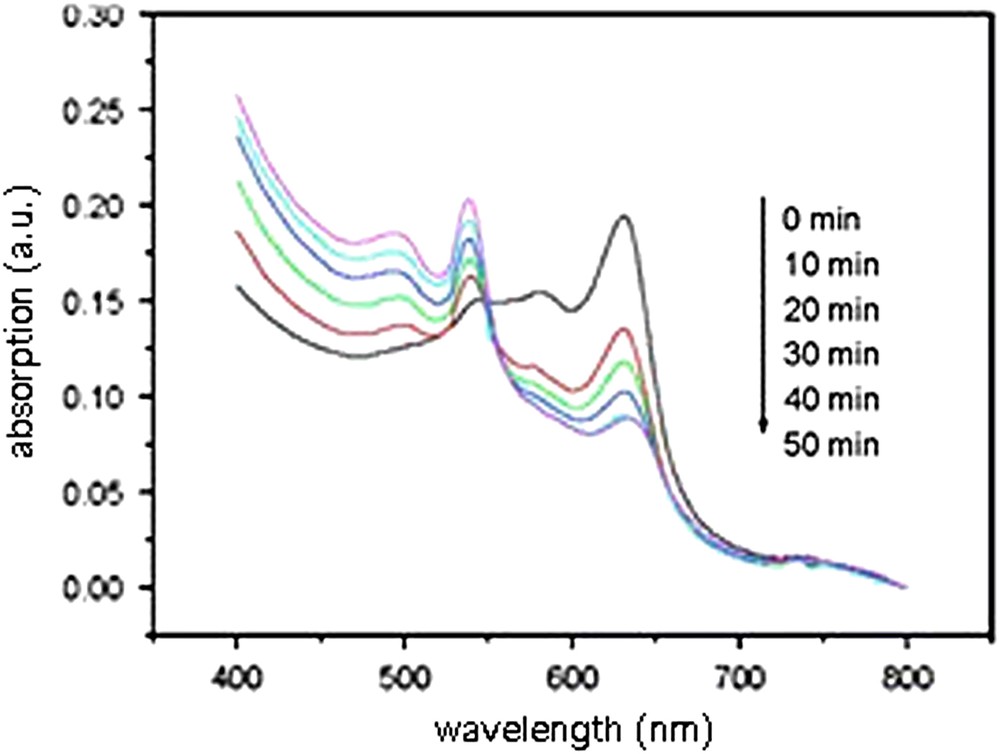
Time dependence of UV–visible absorption spectra of polymerized unextruded vesicles composed of 10,12-PCDA and glycolipid 14b3 (50%) after addition of con A [67].
The color change was then registered as a function of increasing amounts of con A added to unextruded vesicles containing 5% molar 14a3, 14b3 or 14c3. As reported in Fig. 7, the color change was depending on the glycolipid chain lengths, the best response being observed with the C14 alkyl chains (14b3).
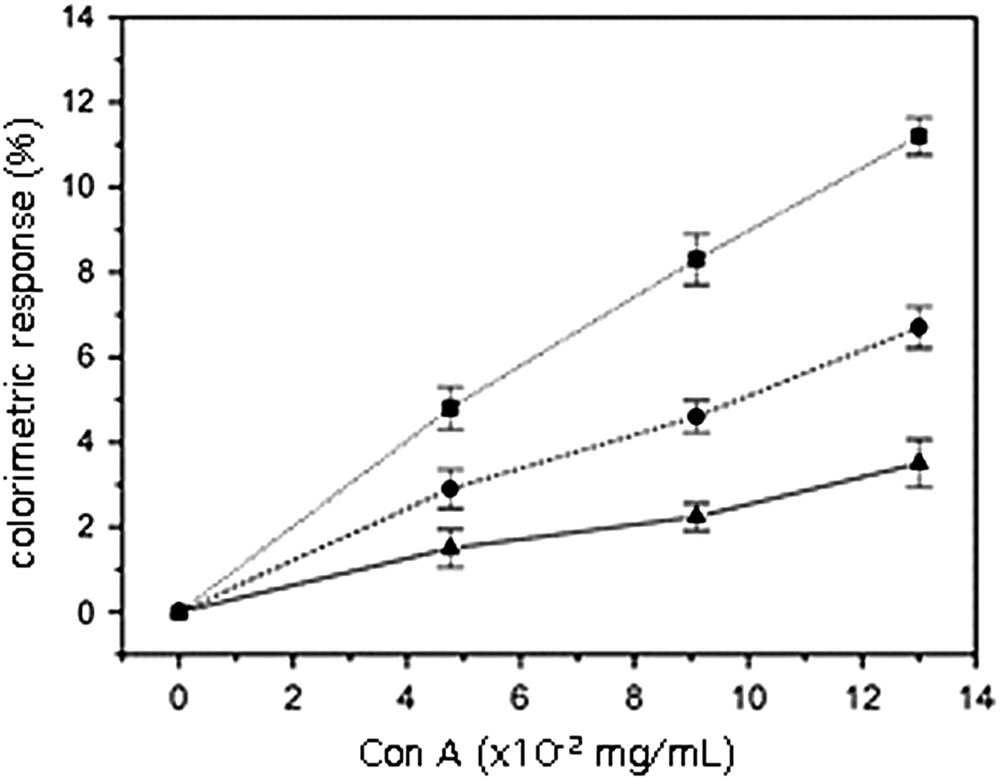
Effect of the glycolipid alkyl chain length on the colorimetric response. Polymerized unextruded vesicles of 10,12-PCDA containing 5% glycolipid: ▴ (14a3), ■ (14b3), ● (14c3) [67].
This result can be interpreted as follows. In the case of C16 alkyl chains, the glycolipid 14c3 was shown to exist in the gel phase, which is unfavourable to molecular recognition. Furthermore, the chain length is favourable to a deep embedment into the polymeric network. In the case of C11 alkyl chains, 14a3 does not exhibit high hydrophobic interactions with the polymerized matrix thus explaining a relatively low disturbance of the latter during molecular recognition by the lectin. In this example, the C14 alkyl chain length represents the best compromise between organization and recognition [67].
Using the glycolipid that gave the best recognition results (14b3), a systematic study was realized with regard to color change versus carbohydrate amount into the polymerized vesicles. As can be seen in Fig. 8, addition of increasing amounts of glycolipid in the polymerized vesicles resulted in an increase of color change in the presence of con A. Nevertheless, the observed effect was not drastic, mostly if compared with the recognition of vesicles of polymerized 10,12-PCDA only. On the contrary, when the vesicles were extruded before polymerization, a drastic increase in the color response was observed, as well concerning its intensity at its speed. For example, unextruded vesicles made of 10,12-PCDA and 14b3 (50%), exhibited 15% color response in 24 min with 10.5 × 10−2 mg/mL of con A. The same response was obtained in 7.5 min and 3.5 × 10−2 mg/mL of con A only, when the vesicles were extruded through 0.2-μm films, before polymerization [67].

Colorimetric response to con A of unextruded (solid lines) polymerized vesicles of 10,12-PCDA containing various amounts of glycolipid 14b3. From bottom to top: ● 0% (pure diacetylenic vesicles), ▶ 5%, ◀ 10%, ♦ 50%. The dashed line (★) is the color response observed for 10,12-PCDA/14b3 (50%) vesicles extruded through a 0.2-μm film. Data recorded after 30 min incubation with con A [67].
A systematic study was realized to rely on the sizes of the vesicles with their molecular recognition by con A. When extruded through nylon membranes of various pore diameters (1, 0.45, or 0.2 μm), vesicles decreased in size and their distribution became narrower, as can be seen in Fig. 9.

Size distribution determined by dynamic light scattering of 10,12-PCDA/14b3 (1:1) vesicles sequentially extruded through nylon membranes. (A) Unextruded, (B) extruded through a 1-μm membrane, (C) extruded through a 0.45-μm membrane, (D) extruded through a 0.2-μm membrane [67].
Before extrusion, vesicle sizes display a wide distribution centred near 650 ± 300 nm; when extruded through 1-μm membranes, the distribution was centred near 140 ± 30 nm, whereas extrusion through 0.45 and 0.2 μm membranes led to narrower distributions of vesicle sizes centred near 85 ± 15 nm and 60 ± 10 nm, respectively.
The influence of the size upon recognition (and color response) was experienced by adding increasing amounts of con A to suspensions of polymerized 10,12-PCDA/14b3 (1:1). As can be seen in Fig. 10, the color response was related to the vesicle sizes: the smaller the size, the better the response.
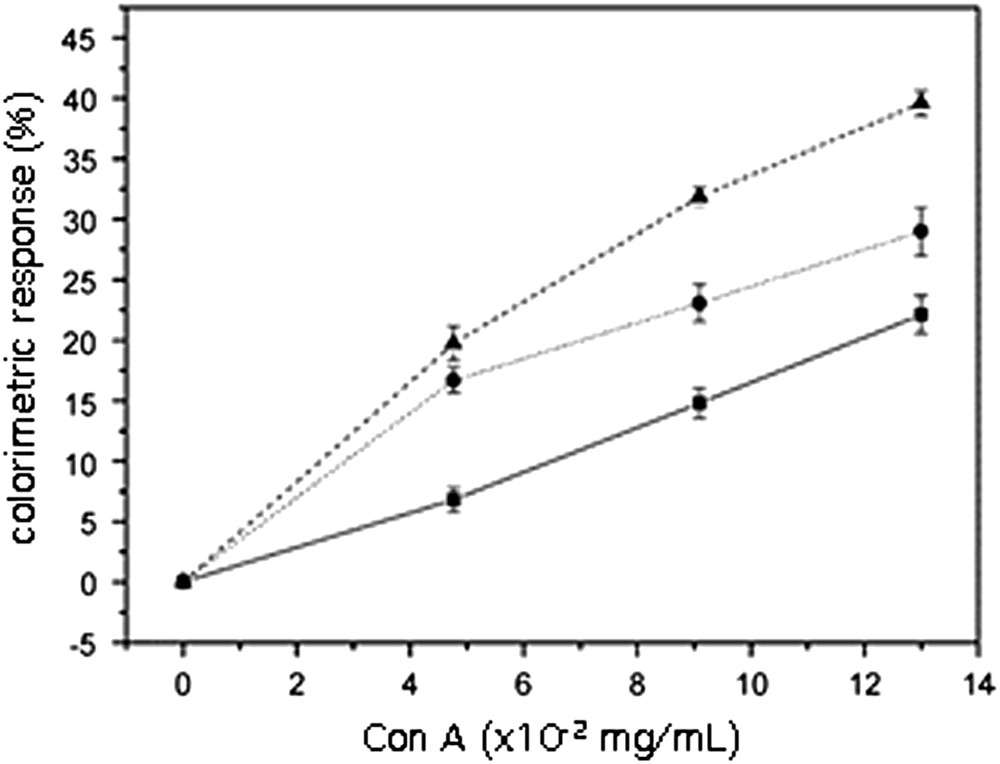
Influence of the membrane pore sizes on the color transition. Vesicles 10,12-PCDA/14b3 (1:1) extruded through ■ 1 μm, ● 0.45 μm and ▴0.2 μm membranes, respectively [67].
An explanation for this observation can be found in the respective sizes of con A and vesicles and in the vesicle curvature. As said previously, the mean diameter of con A is about 6 nm, that of the unextruded vesicles is 650 nm, whereas that of the smallest extruded ones is near 60 nm. Assuming an uniform repartition of the glycolipids at the surface, it could be possible that the approach of the lectin to all the carbohydrates residues is hindered at the surface of the largest vesicles where glycolipids lie almost parallel, whereas the approach could be more favoured when the high curvature of the smallest vesicles imparts a more pronounced angle between the glycolipid molecules. Nevertheless, this explanation, which is reasonable with regards to similar results already reported in the literature [68], remains hypothetical, since other parameters could interfere in the recognition phenomenon, such as the formation of glycolipid clusters or rafts at the interface, the change in the 10,12-PCDA/glycolipid ratio during extrusion, or conformation changes at the interface that could displace monovalent to multivalent binding with the lectin.
2.4 Further developments
It was demonstrated that the addition of phospholipids to the polydiacetylenic backbone was able to increase the color response. Thus, addition of increasing amounts of dimyristoyl phosphatidylcholine to 10,12-PCDA vesicles was able to enhance the color change observed during the addition of a cationic surfactant (cetyltrimethylammonium bromide, CTAB) as reported in Fig. 11 [69]. The ability of CTAB to induce chromatic transition of the vesicles is related to the positively charged head groups of CTAB, which favours approach of the latter to the negatively charged carboxylate and phosphate groups at the surface of vesicles. Also, the color change was very sensitive to pH, as shown by comparison of Fig. 11 (pH 4.0) and Fig. 12 (pH 6.88). Clearly, CTAB adsorbs more onto the vesicle surface when the negative charge is higher. Then, hydrophobic interactions could result from a structural reorientation of CTAB molecules whose alkyl chains could insert into the hydrophobic backbone of the vesicles, thus perturbing the conformation of polymer backbone and leading to color change of the mixed vesicles [69].

Colorimetric response of mixed 10,12-PCDA/DMPC vesicles to increasing amounts of CTAB in water at pH 4.00 and DMPC/PCDA ratios: ▴(3:7), ▾ (5:5) and ♦ (7:3) [69].

Colorimetric response of mixed 10,12-PCDA/DMPC vesicles to increasing amounts of CTAB in water at pH 6.86 and DMPC/PCDA ratios: ▴(3:7), ▾ (5:5) and ♦ (7:3) [69].
The enhancement of color response by addition of phospholipids in the polymeric backbone was used in molecular recognition of glycolipids by E. coli. Thus, when vesicles were prepared from 10,12-PCDA, glycolipid 13 (20% molar ratio with regard to PCDA + DMPC) and various amounts of DMPC, a blue solution was obtained after polymerization upon exposure to UV irradiation at 254 nm. In the presence of E. coli, the solution turned to red as a result of molecular recognition of the maltotriose by the bacteria. The recognition was shown to be strongly dependent on the amount of DMPC in the vesicles and on the pH of the solution [70]. The results are reported in Figs. 13 and 14.
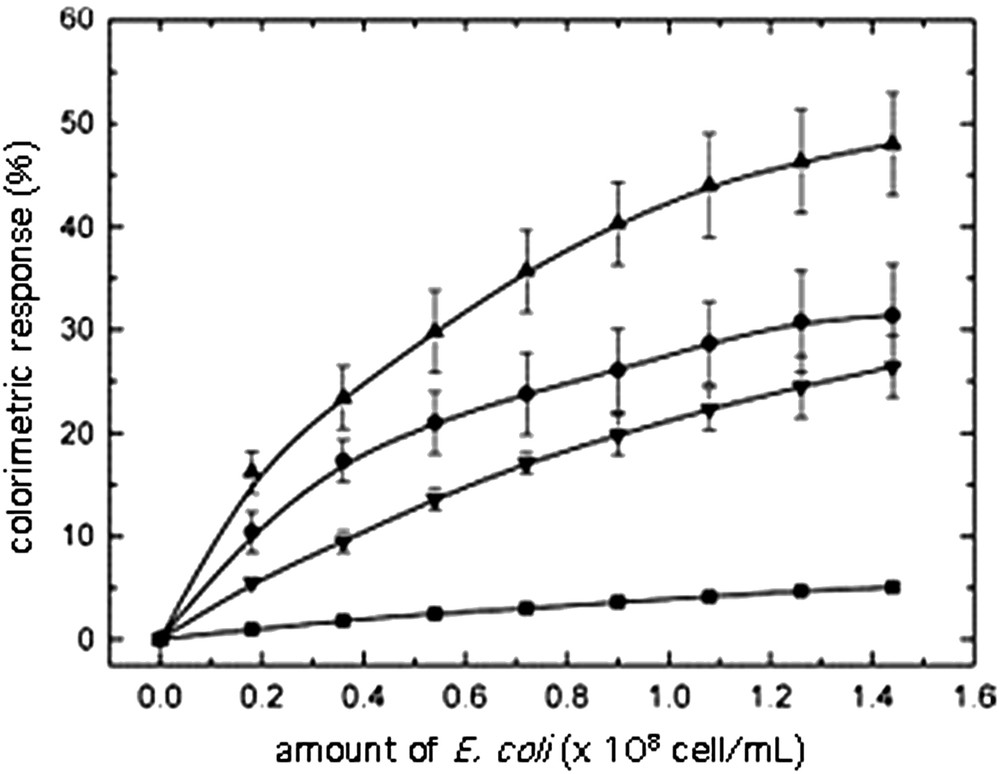
Influence of DMPC content upon the colorimetric response of mixed PCDA/DMPC/13 vesicles as a function of the amount of E. coli. Aqueous solution, pH 8.5, DMPC/PCDA/13 ratios: ■ (0:10:0.2), ● (3:7:0.2), ▴(5:5:0.2), and ▾ (7:3:0.2) [70].

Influence of pH upon the colorimetric response of mixed PCDA/DMPC/13 vesicles as a function of the amount of E. coli (DMPC/PCDA/13 ratio, 5:5:0.2). pH: ■ (7.0), ● (8.0), ▴ (8.5), and ▾ (9.0) [70].
That the color change was effectively due molecular recognition of the carbohydrate by E. coli was attested by the lack of color change of vesicles in which no glycolipids were embedded, or the lack of color change of mixed 10,12-PCDA/DMPC/13 vesicles upon exposure to BSA. As can be seen in Fig. 13, the color change was enhanced by addition of DMPC in the bilayer backbone; this can be due to a decrease of the activation barrier of the colorimetric change. Nevertheless, when the DMPC/10,12-PCDA ratio is >1, the color response is lowered, since more DMPC could alleviate the stress on the adjacent 10,12-PCDA network [70].
The pH was also shown to play an important role in the color change, as can be seen from Fig. 14. An increase of pH induces ionization of the carboxyl groups (ionization changes of phosphates are not effective in the studied pH range), thus inducing some tangling in the polymer backbone that lowers the energy barrier, necessary for the color change. With a view to developing a biosensor in which polymerized vesicles could constitute the color changeable device and glycolipids the sensitive element, it is worthwhile to mention that important increases in signal detection can be obtained by adding various amounts of phospholipids into the vesicles and by adjusting the pH to values that favour ionization at the interface.
Also, it has been established that polydiacetylenic films can undergo distinct fluorescence transitions due to conformational transitions in the conjugated enyne backbone, induced by molecular interactions and surface perturbations (Fig. 15) [71,72]. For instance, Kim et al. [71] have shown that after interaction of polydiacetylene and cyclodextrin, an apparent fluorescence was observed.

A schematic representation of the interaction between polydiacetylene and cyclodextrin [71].
2.5 Detection of WGA by polymerized Langmuir–Schaefer films
As a step to the development of biosensors, based on colorimetric detection, we studied the molecular recognition of β-d-GlcNAc by a specific lectin (wheat germ agglutinin, WGA). For that purpose, monolayers of mixed polymerized films of 10,12-PCDA and glycolipid 14b3 were deposited on glass slides previously covered with n-octadecylsilane (Fig. 16). The films were firstly studied in details, before polymerization. Surface-pressure isotherms of 10,12-PCDA films and various ratios of glycolipid 14b3 (Fig. 17) displayed an increase of stability and an increase of fluidity (compared with pure 10,12-PCDA) when increasing amounts of glycolipid are added to the mixture. The ratio of glycolipid in the monolayer was also shown essential to maintain the minimal distance between the diacetylenic chains for polymerization. A molar ratio of 5% glycolipid was found suitable for polymerization and recognition experiments [71].

Schematic representation of the mixed deposited film of 10,12-PCDA and glycolipid 14b3 on an octadecylsilylated glass slide [73].

Surface pressure isotherms of mixed 10,12-PCDA/14b3 films containing different molar ratios of glycolipid, spread on pure water sub-phase at 25 °C. 14b3/10,12-PCDA molar ratio: (a) 0 (pure PCDA), (b) 0.3, (c) 0.5, (d) 0.7, (e) 1 (pure 14b3) [73].
Several layers could be transferred on the hydrophobized support by Langmuir–Schaefer deposition to form a X-type multilayer; the blue color of the film was visible to the naked eye and its intensity was shown to be proportional to the number of layers transferred, as displayed in Fig. 18. When the coated glass slide was immersed into an aqueous WGA solution, its color turned from blue to red after a few minutes; nevertheless, 2 h were found suitable to reach the maximum color change. The UV–visible absorption spectrum displayed a shift of the maximum intensity from 637 to 543 nm. The specificity of the colorimetric response, attributed to recognition by WGA, was demonstrated by incubation with BSA that induced a very low colorimetric response only (4.6%), by comparison with WGA (43.3%) for the same concentration of protein and the same incubation time [73]. Whereas the intensity of blue color was shown proportional to the number of deposited monolayers, the colorimetric response was found inversely proportional, as seen in Fig. 19. Furthermore, the time required to obtain the maximum answer was longer for a high number of deposited monolayers. These results suggest that the outer layer is mostly responsible for the color change, as it could be anticipated. The molecular recognition is mostly affecting the carbohydrate residues lying at the interface, and the inner layers are not affected and left almost undisturbed.
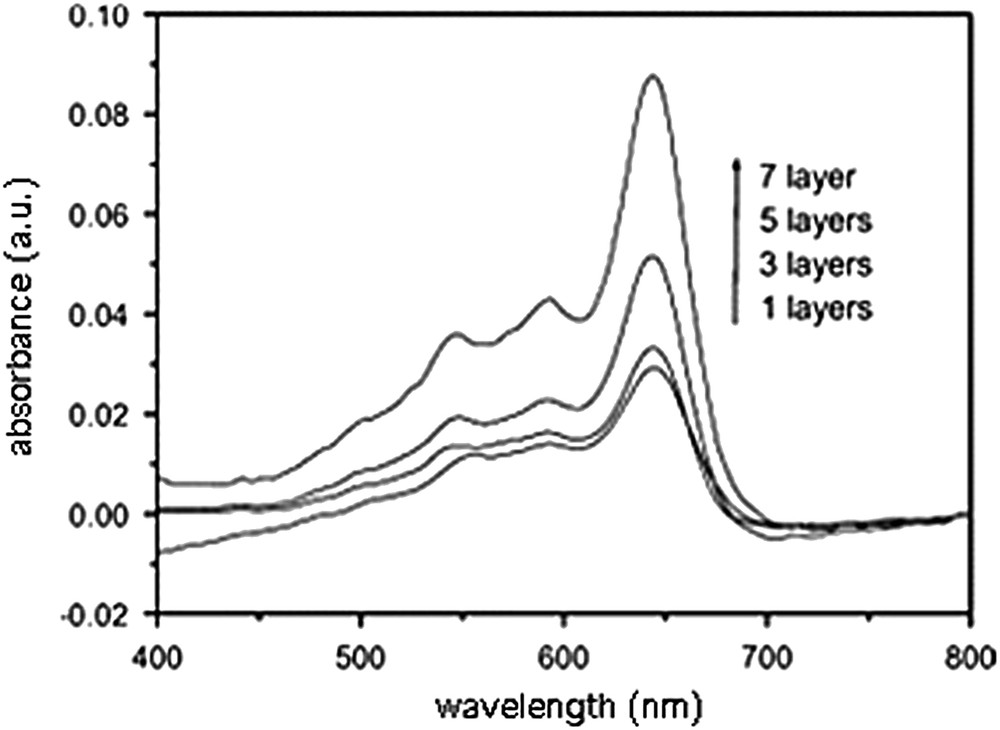
UV–visible absorption spectra of mixed 10,12 PCDA/14b3 Langmuir–Schaefer films, with increasing number of layers [73].
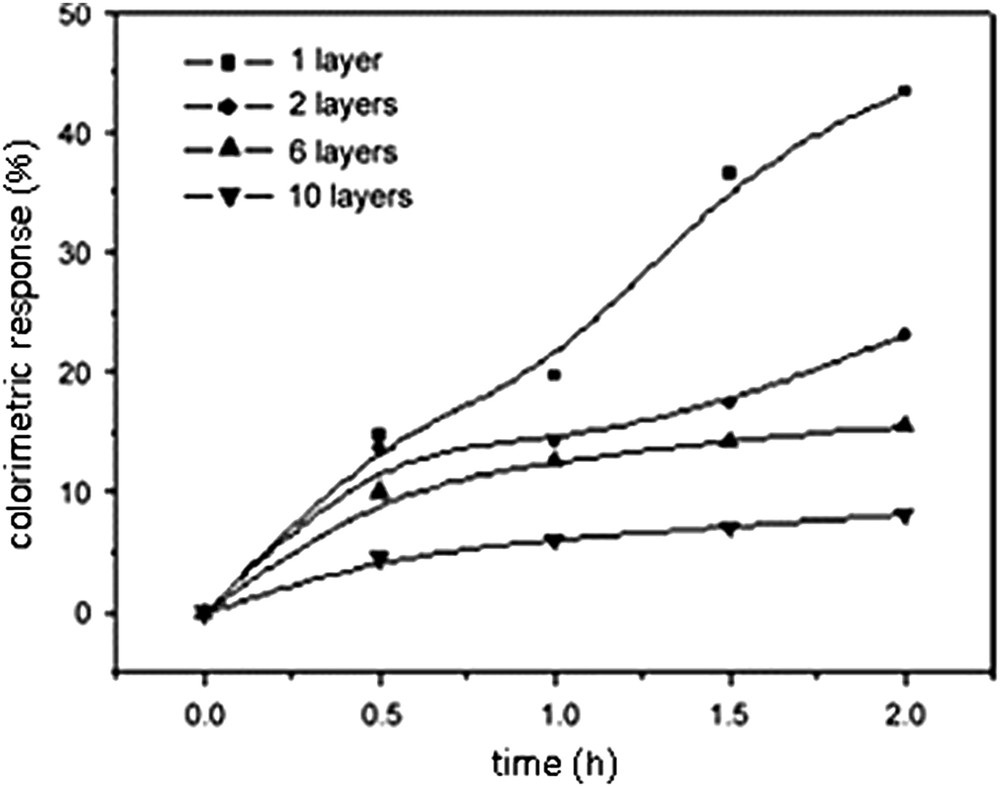
Time dependence of the colorimetric response to WGA of mixed 10,12 PCDA/14b3 Langmuir–Schaefer films with increasing number of layers [73].
3 Conclusion
Synthetic neoglycolipids embedded into polydiacetylenic monolayers or vesicles are able to induce a color change when brought together with a complementary protein or microorganism. Lectins, such as wheat-germ agglutinin (WGA) or concavalin A (con A) and microorganisms, such as E. coli, were shown to be able to induce the color change. The physico-chemistry of monolayers constituted by mixtures of diacetylenic acids and glycolipids gives interesting information with regard to the ideality and stability of the mixed assemblies, which allow optimizing the recognition parameters. In the case of vesicles, the size and the addition of a third constituent (i.e. phospholipid) was also shown to increase the sensitivity of detection to a large extent. A higher pH was also shown to increase the sensitivity of detection.
With all these parameters in hands, we are now able to control the balance between embedment and accessibility of the ligand to optimize the recognition. The synthesis of new neoglycolipids, under investigation, will allow studying the parameters that govern and favour the specificity of recognition, in order to design biosensors, bioreactants or bio-reactors that could serve for diagnosis purposes.
Acknowledgements
This work was supported by the French ‘Centre national de la recherche scientifique’ and the Chinese Academy of Sciences in the frame of an International Scientific Collaboration Program (PICS 2996 and NSFC 90206035 and 20503036).