1 Introduction
Perovskite-type manganese oxides are widely used as catalysts in environmental chemistry that deal with the conversion of carbon monoxide or hydrocarbons [1]. These oxide catalysts possess both high thermal stability and activity and could be useful in the combustion of fuels in automobiles and in power plants. However, the major obstacles to the successful application of these materials in a large scale are both their low resistance to sulphur poisoning and also their scarce BET surface area, which is often linked to catalytic activity [2]. For this, development of more active catalysts has become a challenge to be overcome. Over the years there has been a considerable interest in obtaining a fundamental understanding of sulphur-poisoning phenomena responsible for the low performance of perovskite catalysts in presence of traces of sulphur compounds in the fuels [3]. Useful knowledge in this subject can be obtained through surface analyses techniques which nowadays allow a deeper study of the interaction of molecules with catalytic surfaces (in situ infrared spectroscopy, thermo-programmed reduction, desorption or reaction TPR, TPD or TPRS combined with mass spectrometry, etc.) [4].
Perovskite synthesis can be generally carried out in several ways, among which are the dry process, usually the ceramic method with thermal treatment higher than 1000 °C [5], and the low-temperature synthesis route such as co-precipitation [6–8], sol–gel process [9–12] and the flash combustion synthesis method [13–15]. Thus, besides the inherent advantages of the use of these latter methods, which avoid the excessive losses of BET surface area of the catalysts, a low resistance to sulphur poisoning of the catalysts and a consequent catalysts' activity decrease have been observed [16]. Therefore other preparative procedures of perovskite-type oxides have to be found to increase both BET surface area and sulphur-poisoning resistance.
Hydrothermal processing is a very convenient technique for the preparation of various multi-component oxide materials. These multi-component oxides from electronic ceramic or catalytic applications can be produced by hydrothermal synthesis at moderate temperatures and pressures (>100 °C, 0.1 MPa) [17]. In the last decade, several publications have appeared in literature, devoted to various aspects for preparation of perovskite-type oxides owing to their potential applications in modern technology. While several perovskite-type titanates [18–20], or zirconates [21,22], were prepared successfully under hydrothermal conditions in the presence of microwaves, to our knowledge, no perovskite-type lanthanum manganites were synthesised by this technique. Previously we reported an easy and fast method for the synthesis of La1−xAxMnO3 (x = 0, 0.2; A = Sr or Ag) perovskites at atmospheric pressure by microwave treatment of mixtures of metal nitrates [23]. The main feature of the dielectric heating is that it utilises the inherent properties of the liquids, solids, and their mixtures to convert microwave energy in situ into heat that promotes the reactions.
In the present study, we have reported both a conventional hydrothermal process and a microwave-assisted hydrothermal synthesis route for the preparation of La1−xAgxMnO3+δ (x = 0, 0.2) perovskites. This closed-system technology not only prevents pollution at its source, but also saves time and energy.
The surface physico-chemical properties change, induced by the kind of thermal treatment, i.e. conventional heating or microwave irradiation, has been studied. A correlation between the surface properties of the catalysts and the catalytic activity in methane combustion in the absence and presence of hydrogen sulphide is reported.
2 Experimental
2.1 Synthesis of precursors under hydrothermal conditions (conventional or microwave irradiation)
The synthesis of La1−xAgxMnO3+δ (x = 0, 0.2) perovskites were performed using La(NO3)3·9H2O (Aldrich, 98%), Mn(NO3)3·4H2O (Aldrich, 98%) and Ag(NO3) (RP, 99.8%). The precursors were prepared adding at room temperature, in suitable ratio, nitrates of lanthanum, manganese and silver (when necessary) to a solution of ammonia under stirring. At 25 °C the mixture was maintained at a pH of 8.5 under vigorous stirring until the obtainment of a homogeneous product which was divided into two parts, then transferred into Teflon containers which were collocated inside autoclaves and heated: a Parr autoclave was used for conventional heating, and an advanced Microwave Model Microsynth Labstation was employed for microwave processing. The instrument, operating at 2.45 GHz with a power supply of 1000 W, is a multimode cavity equipped with temperature- and pressure-monitoring device.
While one part of samples has been conventionally heated at 200 °C under 20 atm for 24 h, the other part of samples was treated under microwaves at 200 °C for different periods of time (30–120 min) and power applied (100–1000 W). In both cases the solid products recovered by centrifugation and filtration have repeatedly been washed three times with 150 ml of distilled water and then dried at 110 °C/12 h. The final products were transferred into alumina crucible and treated conventionally at 700 °C for 10 h.
2.2 Characterisation
Thermal decomposition of the precursors, obtained after hydrothermal ageing, was studied under air using a PerkinElmer instrument (Pyris Diamond TG/DTA analyser). The samples, weighing ca. 20 mg, have been placed in a platinum crucible and heated, with a heating rate of 10 °C min−1 up to 900 °C. The gas feed was 60 ml min−1.
X-ray diffractograms were recorded with a Siemens D 500 diffractometer using Cu Kα radiation (λ = 1.54178 Å) operated at 40 kV, 30 mA and a scanning speed of 2° (2θ/min).
La, Mn and Ag amounts present in perovskites were determined using chemical analysis (dissolution + atomic absorption).
SEM analyses were performed using a Hitachi 800 microscope.
Temperature-programmed reduction-mass spectrometry (TPR-MS) or temperature-programmed desorption-mass spectrometry (TPD-MS) experiments were performed in a linear temperature range of 25–1150 °C (heating rate of 20 °C min−1) using 40 mg of samples introduced in a U-shaped quartz reactor and a QMS apparatus (Pfeiffer vacuum). For both experiments a total flow rate of 1.8 L h−1 was used (1% H2 in helium for TPR experiments and pure helium for TPD runs).
X-ray photoelectron spectroscopy (XPS) analyses were performed with a Riber SIA 200 spectrometer (Riber, Rueil-Malmaison, France) using an aluminium (Al Kα = 1487 eV) X-ray source and a take-off angle of 65° with respect to the specimen surface. C 1s peak at a binding energy of 285 eV was used as an internal standard. The core levels of La 3d, Mn 2p, O 1s, and Ag 3d species were recorded and their relative intensities determined.
Catalytic runs were performed at temperatures ranging from 250 to 750 °C using 1% methane, 4% oxygen, 95% N2 and a total flow rate of 7 L h−1. Resistance to sulphur poisoning was evaluated using 100 ppmv of H2S in reactant mixture. The reactor effluents were periodically sampled (each 5 min) and simultaneously analysed on line by using an Agilent micro-chromatograph equipped with high-sensitivity (5 ppm) thermal-conductivity detectors.
3 Results
3.1 Thermal analysis
Fig. 1 shows TGA profiles for microwave and conventionally synthesised precursors (MWhyd-Prec and CHhyd-Prec) previously dried at 110 °C. Both samples reveal initial water loss (ca. 5–7%) followed by two rapid transitions centred, respectively, at 225 and 675 °C and one slow mass loss in the 230–625 °C temperature interval. The weight loss between 25 and 230 °C was ca. 10.4% for microwave-synthesised sample and 14.7% for conventionally prepared material. Both MWhyd-Prec and CHhyd-Prec samples display, respectively, 2.2% and 1.5% loss at 675 °C. Total weight loss for microwave and conventionally synthesised materials was 24% and 28.6%, respectively. The difference of weight loss observed at 675 °C may be due to the increase of Mn mean valence depending on the heating mode used during hydrothermal treatment (conventional or microwave heating). Zhao et al. [24] have previously shown that, for hydrothermally synthesised LaMn2O5, the valence of Mn changed at around 700 °C with change of measurement atmosphere.
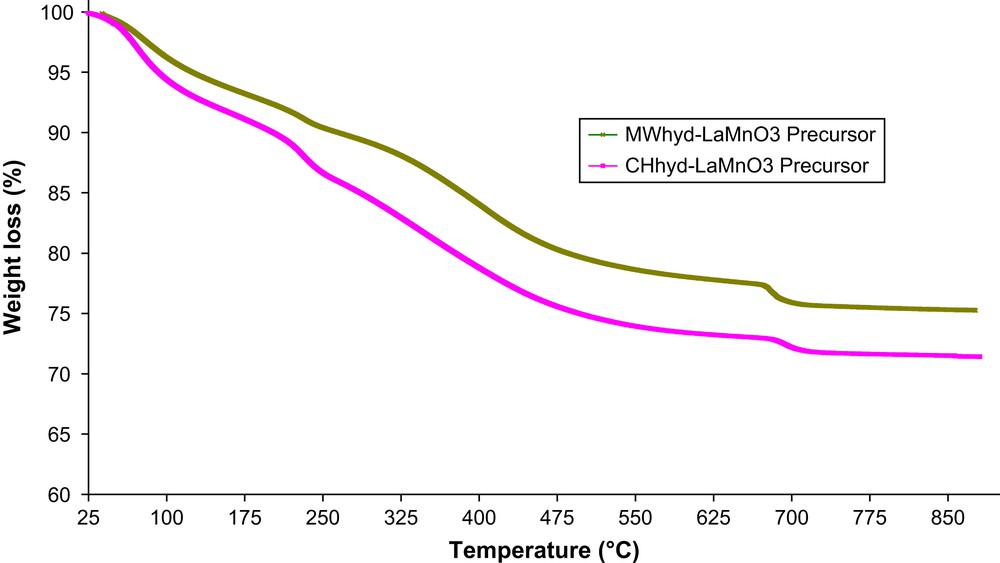
TG profiles for microwave and conventionally synthesised precursors.
3.2 X-ray diffraction analyses
Fig. 2 illustrates the diffraction spectra recorded for all the catalysts synthesised in the present study: it confirms the presence of a crystalline lanthanum manganite phase (JPCDS card: PDF no. 50-297). However, since the detection limit of this technique is 4%, the presence of minor crystalline phases cannot be excluded. Atomic absorption analysis confirmed that the overall amount of the various elements of interest (La, Mn, Ag) was consistent with that used in the precursors and was compatible with the phase detected by X-ray diffraction. To get more evidence of silver doping in the perovskite lattice and to evidence the presence of eventual minor coexisting phases and/or structural defects in lanthanum manganite samples, the neutron diffraction refinement of the crystal structure of La0.8Ag0.2MnO3 [25,26] is necessary: this issue will be investigated in further studies.

X-ray diffraction patterns for the microwave and conventionally synthesized La1−xAgxMnO3+δ (x = 0, 0.2).
The general trend for both microwave and conventionally synthesised La0.8Ag0.2MnO3+δ is that the lattice parameter and cell volume increase with silver ions incorporation in the LaMnO3 host structure. A slight expansion of cell volume (350.85 versus 349.10 Å3) is observed after silver ion insertion in the MWhyd-LaMnO3 host structure.
The bulk composition of the conventionally prepared and microwave-synthesised samples as determined by atomic absorption is given in Table 1, and surface composition measured by XPS along with BET surface area in Table 2. These data indicate that surface chemical composition does not reflect that of the bulk. A marked surface enrichment with silver has been observed for both La0.8Ag0.2MnO3+δ systems. It is worth noting that at a given bulk content of Mn, the highest extent of surface enrichment with lanthanum is observed for the LaMnO3 host structure, and the lowest extent of surface enrichment with lanthanum is characteristic of La0.8Ag0.2MnO3+δ catalysts.
The bulk composition of the catalysts as determined by atomic absorption
Sample | Weight (%) | Catalyst formula | ||
La | Mn | Ag | ||
Fresh CHhyd-LaMnO3 | 56.3 | 23.3 | – | La0.98Mn1.03O3+δ |
Fresh MWhyd-LaMnO3 | 54.0 | 22.7 | – | La0.94Mn1.01O3+δ |
Fresh CHhyd-La0.8Ag0.2MnO3+δ | 49.1 | 22.5 | 8.7 | La0.83Ag0.19Mn0.96O3 |
Fresh MWhyd-La0.8Ag0.2MnO3+δ | 45.3 | 24.1 | 9.8 | La0.77Ag0.21Mn1.0O3 |
BET surface area and XPS data of fresh and sulphur-poisoned catalysts
Catalyst | SBET (m2 g−1) | Atomic surface composition (%) | ||||
La | Mn | Ag | O | S | ||
Fresh CHhyd-LaMnO3 | 31 | 18.4 | 13.9 | – | 67.7 | – |
Fresh MWhyd-LaMnO3 | 29 | 16.6 | 12.6 | – | 70.8 | – |
S-MWhyd-LaMnO3 | – | 9.4 | 13.9 | – | 71.6 | 5.1 |
Fresh CHhyd-La0.8Ag0.2MnO3+δ | 28 | 12.1 | 12.9 | 6.8 | 68.2 | – |
Fresh MWhyd-La0.8Ag0.2MnO3+δ | 27 | 11.4 | 14.3 | 7.6 | 66.7 | – |
S-MWhyd-La0.8Ag0.2MnO3+δ | – | 6.9 | 9.4 | 11.4 | 64.6 | 7.7 |
3.3 SEM analyses
SEM investigations showed (Fig. 3) that conventionally prepared La1−xAgxMnO3+δ (x = 0, 0.2) were less agglomerated powders with fine crystallite sizes, while the corresponding samples prepared in the presence of microwave irradiation present rather a greater particle size ca. 3.5 times bigger.

Scanning electron micrograph showing the morphology of (A) CHhyd-LaMnO3 and (B) MWhyd-LaMnO3 catalysts.
3.4 H2-TPR measurements
Figs. 4 and 5 show TPR profiles of microwave and conventionally prepared La1−xAgxMnO3+δ (x = 0, 0.2). In all cases two main peaks, characteristic of oxygen depletion, were observed: a low-temperature species, named α, reacting in the 300–600 °C range, and a high-temperature one, named β, desorbing at ca. 900 °C [27]. The α peak strongly depends on the concentration of surface oxygen vacancies. In particular, its onset and intensity depend in part on the nature of the metal B of ABO3 structure, but mainly on the degree of substitution of the A ion with an ion with lower valence [28].

TPR profiles of microwave and conventionally prepared LaMnO3.

TPR profiles of microwave and conventionally prepared La0.8Ag0.2MnO3+δ.
A significant shift to low temperatures of the peak characteristic of α-oxygen species was observed for both catalysts when LaMnO3 was doped with silver (439 versus 462 °C for MWhyd and 396 versus 420 °C for CHhyd).
Hydrogen consumption, corresponding to the depletion of low-temperature α-oxygen species, for MWhyd-La0.8Ag0.2MnO3+δ (1.56 mmol g−1) and for CHhyd-La0.8Ag0.2MnO3+δ (1.91 mmol g−1) catalysts was, respectively, ca. 1.7 and 1.8 times higher than that observed for the corresponding LaMnO3 host structure (0.94 and 1.04 mmol g−1, respectively). This therefore indicates that silver strongly affects the oxygen mobility of lanthanum manganites' perovskites. In several papers it was demonstrated that the catalytic activity of ABO3 perovskites in total oxidation reactions is mainly determined by the kind of B-site cations. The kind of trivalent A-site cation does not influence significantly the catalytic activity. Consequently, a partial replacement of a less active A-site ion by another element would normally significantly enhance its functionality; however, if the two elements act in a complementary fashion, then a dramatically improved catalytic property may be achieved.
Concerning high-temperature β-oxygen species, no significant differences were observed between all samples (LaMnO3 or Ag-substituted perovskites synthesised conventionally or under microwave), i.e. the same temperature of ca. 900 °C is observed for the peak characteristic of β-oxygen species.
3.5 Catalytic activity
Fig. 6 shows the results of the catalytic activity tests in methane combustion performed with microwave and conventionally prepared La1−xAgxMnO3+δ (x = 0, 0.2). Methane conversion increased with reaction temperature for all catalysts which appeared to be active for methane total oxidation. While CH4 oxidation over MWhyd-La0.8Ag0.2MnO3+δ and CHhyd-La0.8Ag0.2MnO3+δ started, respectively, at 255 and 300 °C, the complete combustion was attained, respectively, at 700 and 650 °C. The activity of lanthanum manganese oxide catalysts which are related to the preparation method and the amount of the substituting ion decreased in the order MWhyd-La0.8Ag0.2MnO3+δ > CHhyd-La0.8Ag0.2MnO3+δ ≈ CHhyd-LaMnO3 ≈ MWhyd-LaMnO3. However, the activity curve of MWhyd-La0.8Ag0.2MnO3+δ and those of the other catalysts intersects at ca. 525 °C.

Temperature dependence of methane combustion on microwave and conventionally prepared La1−xAgxMnO3+δ (x = 0, 0.2).
CH4 conversion profiles for CHhyd-LaMnO3 and CHhyd-La0.8Ag0.2MnO3+δ were identical in the whole range of temperature, indicating that CHhyd-LaMnO3 catalytic activity in methane combustion was not improved by silver incorporation into the host structure.
3.5.1 Effect of H2S on catalyst performances in methane combustion
Effect of hydrogen sulphide on catalytic activity of microwave and conventionally synthesised catalysts was investigated in the same operating conditions previously adopted, except for temperature, which was fixed at 450 °C. This temperature was chosen for comparison of the perovskites' activity with that of the reference catalyst Pd/Al2O3 [29]. Fig. 7 shows the CH4 combustion profiles when a H2S concentration of 100 ppmv was added to the feed. It is clear that CH4 conversion decreased with time of test for all catalysts. However, the decrease was more drastic for conventionally prepared catalysts than for microwave-synthesised catalysts. While CHhyd-LaMnO3 was inactive only after ca. 30 h testing, MWhyd-LaMnO3 and MWhyd-La0.8Ag0.2MnO3+δ still retained more than 60% of their original activity.

Results of the catalytic activity test towards CH4 combustion when 100 ppmv H2S was added to the feed.
3.5.2 TPD after CH4 combustion in the presence of hydrogen sulphide
TPD profiles of both fresh (La1−xAgxMnO3+δ, x = 0, 0.2) and sulphur poisoned (S-La1−xAgxMnO3+δ, x = 0, 0.2) catalysts prepared under conventional hydrothermal conditions or in the presence of microwave irradiation are presented in Figs. 8 and 9. The striking feature of oxygen depletion from fresh CHhyd-LaMnO3 sample (peak at 660 °C) is highly shifted upon sulphur poisoning towards higher temperatures (T ≥ 750 °C). The same phenomenon was observed for CHhyd-La0.8Ag0.2MnO3+δ. For microwave-synthesised La1−xAgxMnO3+δ (x = 0, 0.2) catalysts, the shift was less important. The peak at 715 °C observed with fresh MWhyd-LaMnO3 sample was shifted to 770 °C for sulphur-poisoned S-MWhyd-LaMnO3 and to 780 °C for sulphur-poisoned S-MWhyd-La0.8Ag0.2MnO3+δ. It can be derived that the oxygen mobility of H2S poisoned S-La1−xAgxMnO3+δ (x = 0, 0.2) conventionally synthesised was strongly decreased, which is in agreement with their low catalytic activity (Fig. 7). It can be inferred that sulphur poisoning proceeds via the formation of stable sulphate/sulphite species. The decomposition of such species can be observed above 800 °C. Atomic absorption analysis of the sulphur poisoned catalysts recovered after TPD experiments indicated that sulphur was still present in the catalysts (ca. 0.3%). Table 2 shows the surface composition of the fresh and sulphur-poisoned catalysts determined by XPS.

TPD profiles both of fresh and sulphur-poisoned CHhyd-LaMnO3 and CHhyd-La0.8Ag0.2MnO3+δ catalysts.

TPD profiles both of fresh and sulphur-poisoned MWhyd-LaMnO3 and MWhyd-La0.8Ag0.2MnO3+δ catalysts.
La/Mn ratio for both CHhyd-LaMnO3 and MWhyd-LaMnO3 was similar (ca. 1.3) and lower for CHhyd-La0.8Ag0.2MnO3+δ (ca. 0.9) and MWhyd-La0.8Ag0.2MnO3+δ (ca. 0.8). This ratio decreased to ca. 0.7 for both sulphur poisoned S-MWhyd-LaMnO3 and S-MWhyd-La0.8Ag0.2MnO3+δ. The amount of sulphur species present in sulphur-poisoned catalysts prepared under microwaves was sensibly the same. These data were in agreement with the slow decrease of catalytic activity (Fig. 7) during tests performed in the presence of hydrogen sulphide.
4 Discussion
It is well known that the interaction of dielectric materials with microwaves leads to what is generally described as dielectric heating. Electric dipoles present in such materials respond to the applied electric field of microwaves. A convenient measure of the heating effect which occurs in an applied field is tan δ = ɛ″/ɛ′, which is the energy dissipation factor and ɛ′and ɛ″ are the real and imaginary parts of the dielectric constant. In materials where the dipoles rotate freely, such as liquid, the rotational frequency of the dipole determines the dissipation of energy from the applied field. The microwave power dissipation per unit volume in a material, P, is dependent upon the total current σ and the square of the electric field E in the sample: P = σ|E2| = (ωɛ0ɛ′tan δ)|E2|, where ɛ0 is the dielectric constant of free space and ω the frequency of the microwaves.
The application of microwave–hydrothermal techniques to process modern materials is a rapidly expanding technique area of research and development. Microwave–hydrothermal method was first developed for the synthesis of zeolites and subsequently hydrothermal work has been directed towards the synthesis of a range of ceramic oxide powders [21,30,31].
Conventional hydrothermal processing usually yields platy, needles, or irregular agglomerated particles, whereas microwave-hydrothermal processing yields solids with agglomerate-free narrow particle size distribution with spherical morphology, which is expected to have better sintering properties.
Komarneni et al. have carried out the synthesis of BaTiO3, SrTiO3, BaZrO3, SrZrO3, PbZrO3 using both conventional and microwave–hydrothermal techniques [22]. The crystal size, morphology, and level of agglomeration of the different ceramic oxides were found to be controlled by parameters such as concentration of the chemical species, pH, time and temperature. Submicron powders have been obtained by microwave heating [21].
The observations made in the present study on catalyst preparation fully support that microwave heating for hydrothermal ageing of precursors was benefit to the synthesis of perovskite-type La1−xAgxMnO3+δ (x = 0, 0.2). Precursors exposed for only 30 min to microwaves under stirring, prior to calcination at 700 °C for 10 h, did not lead to pure perovskite phase. The operating conditions were optimised for the obtainment of pure crystalline phases (XRD). The time of ageing was shortened to 120 min versus one day using conventional hydrothermal treatment. The acceleration of ageing time with respect to conventional heating may result from the microwave coupling with water molecules and manganese precursors due to their high dielectric constants [32–34]. Selective heating could cause unusual morphology of La1−xAgxMnO3+δ (x = 0, 0.2). In addition, oscillating microwave fields may affect the arrangement of perovskite particles. SEM pictures indicate that the powders consist mainly of arranged spheres. La1−xAgxMnO3+δ (x = 0, 0.2) perovskites synthesised by microwave heating seem to be more homogeneous in terms of morphology and size than the corresponding conventional CHhyd materials and have a greater crystallite size (70 versus 20 nm). This is in agreement with TPR data. These factors can influence their performances as catalysts. Microwave-prepared MWhyd-La0.8Ag0.2MnO3+δ perovskite was more effective in methane catalytic combustion than conventionally prepared CHhyd-LaMnO3 and CHhyd-La0.8Ag0.2MnO3+δ. The similarity in crystallographic structure between microwave and conventionally synthesised samples and the great difference in their activity in methane combustion suggests that the nature of methane catalytic combustion active sites in the former is different from that in the latter. The pre-treatment under microwaves would have an effect for promoting their catalytic actions. An important factor for high activity of MWhyd-La0.8Ag0.2MnO3+δ sample should be its greater oxidation ability, which can result mainly from the amount of Mn species present in the catalytic surface (XPS data).
According to catalytic results in the presence of hydrogen sulphide, microwave-prepared materials were also more stable than conventionally prepared ones. The amount and strength of catalytic sites would have an effect on both oxidation of CH4 and H2S. Contrary to microwave-prepared catalysts, conventional materials lose more quickly their resistance to sulphur poisoning when exposed to H2S, which lowers drastically their activity in methane combustion. This is probably due to both the higher amount of α-oxygen species present in CHhyd catalysts and their mobility. A strong interaction at low temperature between hydrogen sulphide and these species leads to fast sulphur poisoning of the catalysts, which proceeds via the formation of stable sulphate/sulphite species.
Furthermore, we should consider the influence of Mn/La ratios (Table 2). The presence of H2S in the feed during methane catalytic combustion modifies the atomic surface composition of the catalysts, which affects the observed catalytic activity in the absence of sulphur-containing compounds. MWhyd-LaMnO3 and MWhyd-La0.8Ag0.2MnO3+δ (with Mn/La of ca. 0.8 and 1.25 for fresh catalysts and 1.5 and 1.4 for sulphur-poisoned catalysts, respectively) have been found to be more resistant against sulphur poisoning than CHhyd catalysts. The MWhyd catalysts contain fewer amounts of α-oxygen species with lower mobility that is reflected in the higher temperature of oxygen depletion (ca. 55 °C higher than the corresponding CHhyd catalysts).
From correlations between catalytic behaviour and physico-chemical properties of La1−xAgxMnO3+δ (x = 0, 0.2) it appears that the use of microwave irradiation for the synthesis of perovskites leads to catalysts with high oxidation potential, very active for CH4 and H2S combustion and resistant against sulphur poisoning.
5 Conclusions
In this work, we examined the activity and stability of La1−xAgxMnO3+δ (x = 0, 0.2) catalysts for methane combustion in the presence and absence of H2S. Perovskites were synthesised conventionally and in the presence of microwave irradiation. Microwave heating during the hydrothermal ageing step has a pronounced effect on physico-chemical and catalytic properties of the catalyst. The microwave-synthesised MWhyd-La0.8Ag0.2MnO3+δ catalyst demonstrated excellent conversion of methane. Furthermore, microwave-synthesised catalysts remained active in the presence of hydrogen sulphide, while conventionally prepared catalysts deactivated quickly. The superior catalytic performances of microwave-prepared perovskites seem to arise from their atomic surface composition, lower amount of α-oxygen species and their moderate mobility. Thus, the potential of improving the activity of perovskite-type catalysts using the hydrothermal assisted-microwave method is very promising.