1 Introduction
Dendrimers [1] undoubtedly constitute one of the major fields of research connecting chemistry to nanosciences, with more than 1000 publications and more than 100 patents per year since five years. These very special types of polymers, hyperbranched and multifunctional, elaborated step by step (generation after generation), constitute a particular example of “soft matter” [2]. Thanks to the numerous properties they possess in various fields such as catalysis, materials and biology, interest in dendrimers is continuously growing each year. Macrocycles [3] are another type of macromolecules that have also attracted the attention of researchers in different fields for several decades now. Combination of both types of macromolecules is supposed to increase the level of preorganization and might induce cooperative effects affording specific properties different from those produced by the separated parts [4]. The first example in this field dates back to 1992 [5]; it concerned a dendrimer bearing azacrown ether macrocycles as constituents of each branch (type A in Fig. 1). Such types of dendritic architectures are still relatively rare, but other types of dendrimers functionalized by crown ethers at some of the internal layers (type B) [6], or at each layer [5,7] have also been synthesized, affording brilliant skills as electron carriers. Other structures comprising a combination of porphyrins at each layer of the dendrimers have light-harvesting properties [8], whereas a dendrimer possessing azathia macrocycles at each layer was able to complex palladium [9].

Schematized types of molecular architectures possessing both macrocycles and dendritic branches.
However, the largest number of dendritic structures connected to macrocycles possesses a macrocycle as core (type E, Fig. 1). Porphyrins [10], phthalocyanines [11], cyclams [12], or crown ethers [13], are the types of macrocycles the most widely used for this purpose. A shielding effect of the branches towards the core is generally expected. Dendrimers bearing macrocycles at the periphery are also well known in the literature even though less examples are described than for the macrocycle at the core. It is believed that increasing the number of macrocycles in a single molecule could enhance the properties of such assemblies (type F). Examples with porphyrins [14] and phthalocyanines [15] as peripheral substituents were in particular reported. Fig. 1 displays the various types of molecular architectures connecting macrocycles to dendrimers that have been synthesized up to now. As indicated above, the largest number of examples known to date pertains to types E and F.
In this account, we will describe the specific contribution of phosphorus-containing dendrimers to this field of research. Depending on the location and the type of macrocycles, these compounds possess various properties and uses that will be also described.
2 Synthesis and properties of phosphorus dendrimers including macrocycles
We have a longstanding interest and experience in the synthesis and study of properties of phosphorus-containing macrocycles [16] and dendrimers [17], and we tried very early to combine both types of compounds. The first example of such combination concerns a compound possessing a central macrocycle, functionalized by four linkers bearing at their end another type of macrocycle [18]. The central macrocycle is synthesized using our classical [2 + 2] condensation method between two equivalents of a dialdehyde such as 1 and two equivalents of a phosphodihydrazide such as 2, to afford the 36-membered ring 3. The presence of four azide groups allows performing Staudinger reactions with four equivalents of the phosphine 4 bearing a crown ether. The multimacrocyclic species 5 obtained after a spontaneous rearrangement contains one phosphorus macrocycle at the core and four crown ether macrocycles on the periphery, linked by PNPS linkages, which also constitute the branches of several types of our phosphorus-containing dendrimers [19].
This first example pertains to the type C of Fig. 1, which is relatively rare, but we have also described a very original example of type D compound; such compound is the only example ever described to date for any type of dendrimers in which macrocycles are linked to the internal branches, but do not pertain to the branches.
Our main method of synthesis of dendrimers consists in the repetition of substitution reactions of 4-hydroxybenzaldehyde in basic conditions on PCl2 end groups, followed by the condensation reaction of the aldehydes by the phosphorhydrazide H2NNMeP(S)Cl2, starting from either P(S)Cl3 (a series) [17] or N3P3Cl6 (b series) [20] as core (Scheme 2). Each time these two steps are repeated a new generation is created (the generation number is noted n). Depending on the step considered, these dendrimers are terminated either by aldehydes (series 6-Gn) or by P(S)Cl2 groups (series 7-Gn), both being particularly suitable for further functionalizations to afford the desired properties [21].

Main method of synthesis of phosphorus-containing dendrimers, starting form either P(S)Cl3 or N3P3Cl6 as core.
This method is very powerful (both steps are quantitative) and allows numerous changes. For instance, to obtain the compound of type D shown in Fig. 2, a dendrimer possessing PN–PS linkages in the internal structure such as 8-G3 must be first synthesized. This linkage [19] is obtained by Staudinger reactions used as one alternative step during our classical synthetic process; it is able to undergo a selective alkylation on sulphur, which induces a weakening of the phosphorus sulphur bond. Using a nucleophilic phosphine such as P(NMe2)3 induces a desulphurization and affords tricoordinated phosphorus atoms inside the structure. These PIII are able to undergo a Staudinger reaction, in particular with a phosphorus azide linked to two aldehydes, to afford compound 9-G3 [22]. The presence of internal aldehydes allowed the development of a large number of reactions [23], in particular the condensation with 4-aminobenzo-15-crown-5 10, to afford the very special dendritic species 11-G3 (Scheme 3, Fig. 2) [24].

Expanded structure of the unique example of a dendrimer possessing macrocycles linked to the internal branches (type D of Fig. 1).
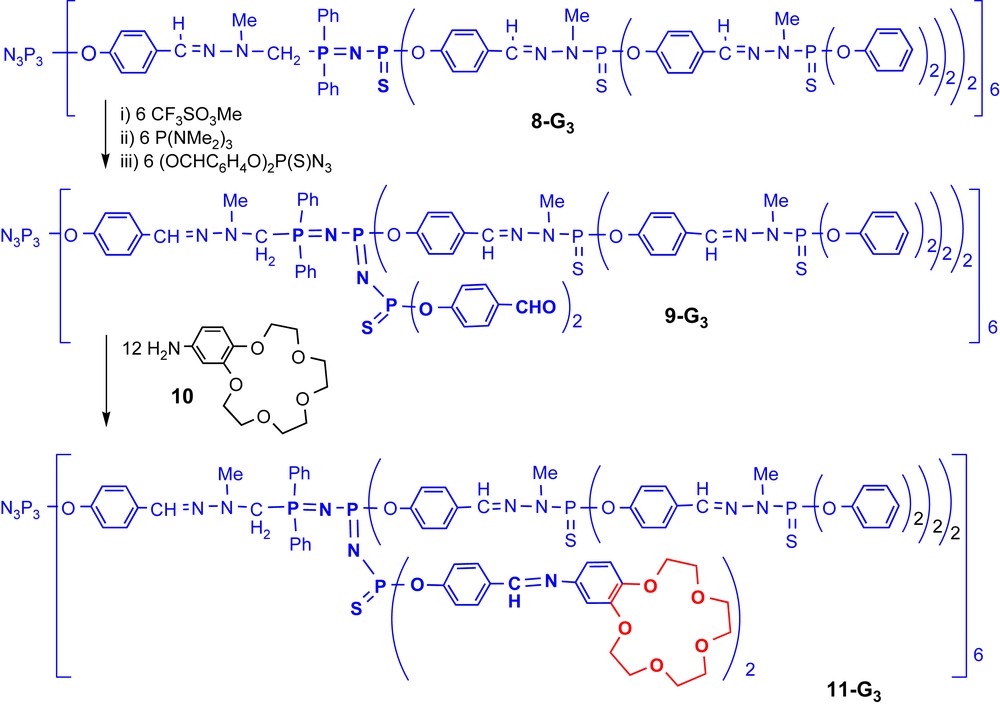
Synthesis of a dendrimer incorporating 12 crown ethers in its internal structure.
Dendrimer 11-G3 demonstrates that the art of synthesis of dendritic molecules can attain a high degree of sophistication, but in most cases we have synthesized simpler dendritic compounds bearing macrocycles either at the periphery (type F) or at the core (type E, Fig. 1).
2.1 Macrocycles at the periphery of phosphorus dendrimers
We have described the synthesis of type F dendritic structures starting from either P(S)Cl2 functions or aldehydes, that are the classical functional terminal groups in our main method of synthesis of dendrimers (see Scheme 2).
We have already shown that the P(S)Cl2 terminal groups of dendrimers 7a-Gn are able to react with primary and secondary amines [25]. By treating dendrimers 7a-Gn (up to generation 3) with three types of macrocycles 1,4,8,11-tetraazacyclotetradecane (cyclam, 12), 1,4,8,12-tetraazacyclopentadecane (13), and 1,4,8,11-tetraazacyclotetradecane-5,7-dione (14) in the presence of K2CO3, we might expect either the grafting of one or two macrocycles per P(S)Cl2. In the case of a single macrocycle, the grafting can lead either to 5-membered rings (diazaphospholanes) or 6-membered rings (diazaphosphorinanes) starting from 12 and 13, whereas only 6-membered rings can be obtained in the case of 14, due to the known low reactivity of the amides. In fact, in all cases, only one macrocycle is grafted per P(S)Cl2 group. More surprisingly, the reaction of macrocycles 12 and 13 is highly regiospecific, and only 5-membered heterocycles are created in both cases, leading to dendrimers 12-Gn and 13-Gn (n = 0–3); 6-membered heterocycles are obtained as expected in the case of 14, leading to dendrimers 14-Gn (Scheme 4) [26].
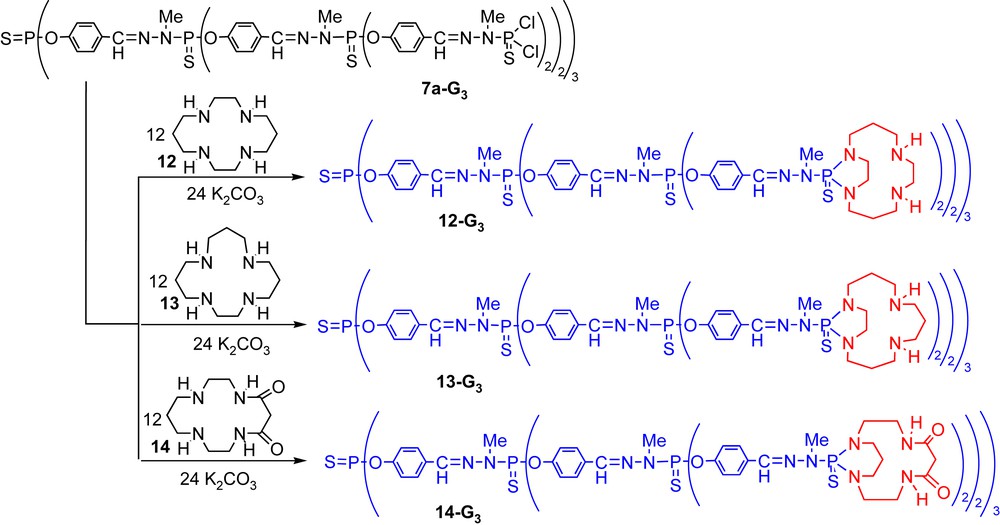
Grafting of various tetraazamacrocycles on the surface of third generation dendrimers.
Starting from the aldehyde terminal groups, the simplest way to graft macrocycles consists in condensation reactions with macrocycles functionalized by an amine. For instance, condensation reactions between the aldehyde groups of dendrimers 6a-Gn (generations 1–4) and 6–48 equivalents of the amino crown ether 10 afford dendrimers 15-Gn, capped by the crown ethers (Scheme 5) [27]. Such bulky substituents necessitate a prolonged time of reaction; completion of the condensations is accomplished within 1 week for the first generation, but necessitates 3 weeks for the fourth generation. Remarkably, terminal crown ethers act as a shield against hydrolysis: the imine functions remain intact after stirring compound 15-G4 for 48 h in a THF/water (80:20) solution. The second generation, possessing 12 crown ether terminal groups, is able to complex 12 equivalents of sodium tetraphenylborate.

Grafting of crown ethers to the surface of a fourth generation dendrimer.
Very recently, we have applied the same method to react the aldehyde terminal groups with the 15-membered triazatriolefinic macrocycles 16 and 17, functionalized by one primary amine. This reaction was carried out with dendrimers 6a-G0, 6b-G0, 6a-G1, 6b-G1, and 6b-G4. Regiospecific reduction of the imine functions by NaBH3CN (the hydrazone functions do not react) ensured the stability and afforded dendrimers 16a,b-G0, 17a,b-G0, 16b-G1, 17b-G1, and 17b-G4 (Scheme 6) [28]. The latter is capped by 96 triolefinic macrocycles.

Synthesis of dendrimers ended by triazatriolefinic macrocycles. This reaction is shown here on a first generation, but was applied up to the fourth generation.
These types of macrocycles are known to be able to complex Pd0 in their cavity [29], thus we tried the same type of reaction with these dendritic compounds. Reactions were attempted with Pd(PPh3)4 and Pd2(dba)4 (dba = dibenzylidene acetone) as sources of Pd0. Depending on both the source of palladium and the nature of the dendritic species (core, generation, substituents of the macrocycles), either discrete palladium complexes or nanoparticles are obtained. A cooperative effect is observed in the case of the fourth generation. Indeed, the ratio macrocycle per Pd can be deduced from elemental analyses: the ratio is 2.4 macrocycle/Pd with the first generation (17b-G1) whereas it is 0.4 macrocyle/Pd with the fourth generation (17b-G4). These nanoparticles have generally a small size (diameters from 2.4 to 5.7 nm), and a narrow size distribution (±1.0 nm). The catalytic properties of the discrete complexes and of nanoparticles elaborated with dendrimers from generation 0 and 4 were tested in Heck reactions. As shown in Fig. 3, in the initial experiments (run 1), the worst results are obtained with the nanoparticles obtained with the fourth generation dendrimer. Recycling experiments were carried out four times in all cases. Surprisingly, the efficiency is improved when the number of recycling is increased. This improvement could be explained by a decrease in the size of the nanoparticles observed by comparing the TEM images obtained initially and after the fifth run [28].

Percentage of conversion in the Heck reaction shown above after 4 h (hollow marks) and after 24 h (plain marks) using 2% of Pd catalyst (either the discrete Pd complex [17a-G0, Pd3] (triangles) or the Pd nanoparticle complexes [17a-G0, Pd7] (squares) or the Pd nanoparticle complexes [17b-G4, Pd238] (rhombus)). After the initial run (number 1), all catalytic samples were reused four times (runs 2–5).
Other types of reactions can be applied to aldehyde terminal groups, and particularly Wittig reactions. The macrocyclic TTF derivative 18 reacts with dendrimer 6a-G4, to afford the fourth generation dendrimer 18-G4 (Scheme 7). This very special macrocyclic dendritic system is able to respond electrochemically, especially when interacting with a guest ion, and is potentially usable as chemosensor. The electrooxidation of dendrimers 18-Gn compares well with that of the monomeric species, whereas the electrodeposition onto the electrode is favoured with the highest generations. The most remarkable feature of these dendrimer films is their unique capacity to control the complexation/expulsion properties of Ba2+ cations. Fig. 4 shows the electrochemical response of the electrode modified by dendrimer 18-G4 when increasing quantities of barium are added [30].

Wittig reactions for the grafting of TTF–crown ether derivatives on the surface of a fourth generation dendrimer.

Modification of the electrochemical response of an electrode covered by electrodeposition of dendrimer 18-G4, when exposed to increasing amounts of Ba2+.
2.2 Macrocycle at the core of phosphorus dendrimers
Two different strategies can be envisaged to obtain a dendrimer possessing a macrocycle as core (type E in Fig. 1): either the classical method of synthesis of dendrimers is applied starting from this particular core or dendrons are grafted to the macrocycle in the final step. Dendrons, also called dendritic wedges, are a special type of dendritic molecules possessing one functional and reactive group at the core, besides the functional groups located at the surface, and are particularly useful to build sophisticated dendritic architectures [31]. We have in particular synthesized a series of dendrons possessing an activated vinyl group at the core, elaborated from vinyl phosphine, and grown using the method of synthesis shown in Scheme 2; these dendrons are able to react with primary and secondary amines in Michael type additions [32]. One example of such dendrons (20-G2) is shown in Scheme 8.

Grafting of dendrons by their core to a nanolatex functionalized by cyclams. The dendron 20-G2 was elaborated from compound 19, by using the method shown in Scheme 2, followed by the condensation of the Girard T reagent to the surface in the last step.
Aqueous suspensions of polymer nanoparticles, also called nanolatexes have a potential wide range of applications, due in particular to their high surface-to-volume ratio. Thus, improved stability and tailored functionalities are a challenging endeavour. Polycationic dendrons of type 20-Gn (n = 0, 1, 2) react readily with the cyclam linked to the surface of nanolatexes (21, diameter 16 nm) (Scheme 8). Remarkably, the number of dendrons linked to the nanolatex depends on the generation (300 20-G0, or 150 20-G1 or 90 20-G2), whereas the number of cations per nanolatex is almost constant (600–800 ammonium groups per particle, whatever the generation used). In all cases, an improved stability of the aqueous suspension is observed, as well as an increased thermal stability. Furthermore, despite the presence of the dendritic layer, the macrocycles at the core retain their metal-complexing ability, with a Cu(II) binding capacity of 0.2 mmol/g of nanoparticle, demonstrating the permeability of the dendritic shell [33].
Another example of grafting dendrons to a macrocycle allowed us to demonstrate for the first time the properties of dendrons as topological amplifiers. Indeed, even if the presence of diastereoisomers in macrocycle 3 (synthesized in Scheme 1) could be anticipated, with the zero value found for its optical rotation strongly suggesting a 50:50 ratio for the stereoisomers on each phosphorus atom, the existence of such diastereoisomers was never detected previously. Even the grafting of hindered but small substituents did not allow to detect any trace of diastereoisomers. On the other hand, the grafting of dendrons through a Staudinger reaction with Ph2PC6H4CHO followed by condensation reactions with the core of dendron 23-G1 affords the dendrimer 24-G2 (Scheme 9). The 31P NMR spectrum of this compound gives sharp signals for the phosphorus atoms pertaining to the dendrons, but multiple signals for the PN–PS linkages directly linked to the macrocycle, instead of the doublets observed with smaller substituents. The minimization of interactions between the end groups of the dendrons necessitates modification of the angles at the level of their roots, differently depending on the diastereoisomers to which they are linked. Since it is known that 31P NMR chemical shifts are very sensitive to modification of the angles around phosphorus, one may attribute the phenomenon observed here to diastereoisomers, demonstrating as expected the role of dendrons as amplifiers of local phenomena [34].

Synthesis of a compound possessing five macrocycles.

Grafting of four dendrons by their core to a 36-membered tetrafunctional macrocycle.
The second strategy usable to obtain dendrimers possessing a macrocycle as core necessitates a multifunctional macrocycle, whose functions are compatible with our classical method of synthesis of dendrimers shown in Scheme 2. The phthalocyanine 25-G0 possesses eight aldehyde groups, thus it is perfectly suitable to react first with the phosphorhydrazide H2NNMeP(S)Cl2, then with hydroxybenzaldehyde in basic conditions, and to repeat several times both steps to continue the growing to afford dendrimers 25-Gn (CHO terminal groups) and dendrimers 26-Gn (P(S)Cl2 terminal groups) (Scheme 10) [35].
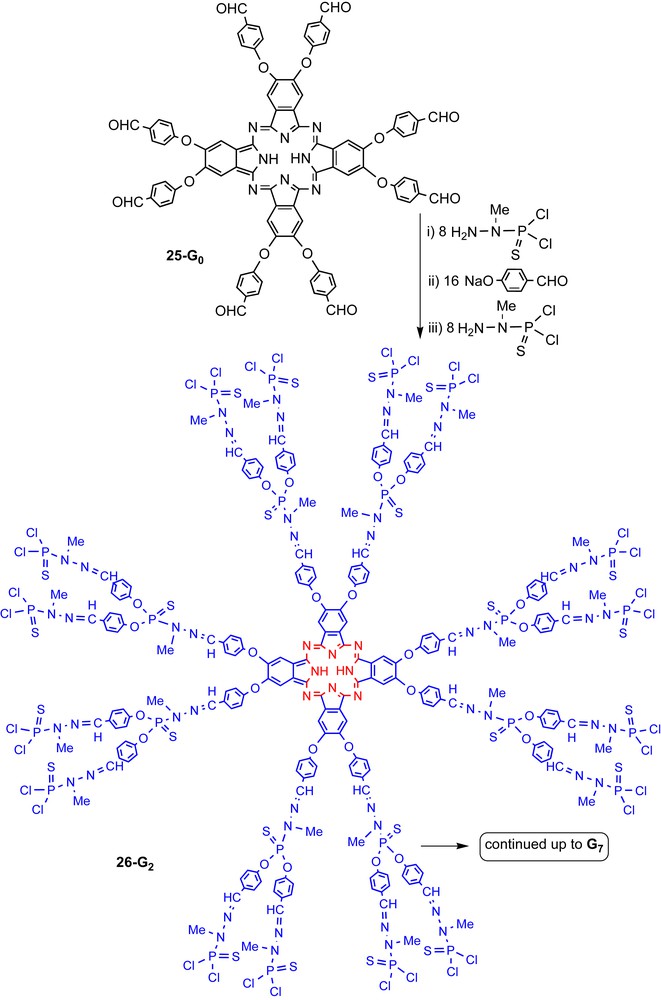
First steps of the synthesis of the series of dendrimers possessing a phthalocyanine as core. The synthesis has been carried out up to the seventh generation, using the method of synthesis shown in Scheme 2. Compounds 25-Gn have aldehyde end groups, compounds 26-Gn have P(S)Cl2 end groups.
The phthalocyanine at the core is usable as a sensor and a probe for determining the properties of the internal structure and the reciprocal influence of the core, branches and surface on the whole structure. Indeed, the UV–vis spectra display both a hyperchromic effect (exaltation of the intensity, due to an increased isolation) and a bathochromic effect (displacement of the wavelength towards the red) on the Q-bands with increasing generations, as well as the disappearance of aggregation phenomena (Fig. 5). Depending on the solvents used these phenomena are more or less pronounced and allowed to demonstrate that the influence of the dendritic branches upon the core mimics a highly polar solvent such as DMF. Measurement of the fluorescence quantum yield shows that it is higher for 25-G3 and 25-G4 than for 25-G0, illustrating an increased site isolation. However, the core remains accessible in all cases, as shown by the addition of NaOH, which induces a dramatic diminishing of the fluorescence intensity, due to the chemical modification of the core when it becomes dianionic with a modified geometry (from D2h for the neutral form to D4h for the dianionic form) (Fig. 6) [35]. The phthalocyanine can also be used to trap metals, in particular copper and cobalt cations. In both cases, the presence of a paramagnetic metal at the core perturbs the NMR response not only of the core but also of the branches at least for the first generations. Furthermore, the total disappearance of the fluorescence upon complexation is observed for all generations [36].

Evolution of the Q-bands of dendrimers 25-Gn with the generation.

Variation of the fluorescence spectra of the generations zero and four of the dendrimers 25-Gn upon addition of NaOH, which induces a diminishing of the band intensity.
The fifth generation of the dendrimers possessing a phthalocyanine as core has been functionalized by ammonium terminal groups, directly obtained by the reaction of NH2CH2CH2NEt2 with the P(S)Cl2 terminal groups of dendrimer 26-G5. This diamine ensures the solubility in water of dendrimers capped by these groups, very useful for various applications related to material science and biology [37]. The grafting of these ammonium groups to 26-G5 affords compound 27-G5 (Fig. 7), which possesses very original properties at the nanometric level. Surprisingly, the presence of a hydrophobic interior and a hydrophilic surface afford in the UV–vis spectra in water the Q-bands characteristic of a phthalocyanine in the solid state. Furthermore, only the signals corresponding to the ethyl (external) groups are detected by 1H NMR, also in water. Both phenomena evoke a frozen internal structure surrounded by solubilizing functions, reminiscent of a solid colloidal state in solution. A progressive addition of THF to the aqueous solution indicates a progressive solubilization of the core, shown by a sharpening of the signals of the Q-band in the UV–vis spectra, and the re-appearance of all the signals of the internal layers in 1H NMR. Diffusion measurements by pulsed-field gradient spin-echo NMR allowed us to deduce the variation of the hydrodynamic radius, which displays a dramatic increase when adding THF, as shown in Fig. 7. In view of these modifications, the internal structure of 27-G5 could act as a nanosponge for lipophilic drugs; we have observed this phenomenon by 1H NMR for the inclusion of tetramethylsilane (TMS). The increase of the volume has also unexpected macroscopic consequences on the viscosity of mixtures water/THF, with 27-G5 acting as a buffer for regulating the viscosity [38].

Variation of the hydrodynamic radius of the water-soluble dendrimer 27-G5 in water with increasing amounts of THF measured by diffusion NMR, and “artist” view of the swelling and blooming phenomena.
As indicated above, water-soluble dendrimers may have interesting biological properties. Compound 27-G5 shows rather moderate cytotoxicity towards Human epithelioid cervical carcinoma cells (HeLa), Human transformed primary Embryonic Kidney cells (HEK 293), and Human Umbilical Vein Endothelial Cells (HUVEC), generally lower than with lipofectin. For instance, the percentage of living HUVEC cells after 48 h in the presence of 10 μg/mL of lipofectin or 27-G5 is ca. 70 and 90%, respectively. Furthermore, this compound is able to act as transfection agent, to deliver fluorescein-labeled oligodeoxyribonucleotide into HeLa cells. The presence of the phthalocyanine as core allowed us to detect the penetration inside the cells, detected by UV-induced fluorescence. The image by fluorescence microscope (Fig. 8) shows that the dendrimer alone is efficiently taken by the HUVEC cells [39], presumably via an endosomal uptake [40].

The effect of incubation for 18 h of dendrimer 27-[G5] with HUVEC cells in the presence of serum, detected by fluorescence microscopy.
3 Conclusion
In this short review, we have shown that molecular design has allowed the synthesis of compounds possessing macrocycles in various parts of dendritic structures, particularly in the case of phosphorus derivatives. Some of these structures, the most original, are only obtainable up to date using the chemistry of phosphorus. If the design of such compounds was made in some cases mainly for aesthetic purposes, it turned out that most of these compounds possess original and interesting properties, arising from the presence of both the macrocyclic and the dendritic parts. The reciprocal influence of both components is particularly obvious when the macrocycle (especially a phthalocyanine) is used as core of the dendrimer. Indeed, the branches act as a shield, preventing in particular aggregation, and partially protecting the core against water, both effects favouring an increased fluorescence, useful when using it as fluorescent probe for biological properties. On the other hand, the presence of a paramagnetic metal trapped by the core disturbs the NMR response of the whole dendritic structure. The presence of a large number of macrocycles on the surface of a dendrimer induces cooperative effects, demonstrated in particular in the case of dendrimers possessing triolefinic triazamacrocycles as terminal groups. Indeed, the stabilization of Pd nanoparticles necessitates a lower number of macrocycles (six times less) when they are connected to a fourth generation than when they are connected to a first generation. All these findings illustrate the highly specific properties of compounds combining these two types of entities (macrocycles and dendrimers) having so particular topologies. Future potential applications of such types of systems can be foreseen in the fields of catalysis, material science, and biology.
Acknowledgements
Thanks are due to ITAV (Institut de Technologies Avancées en Sciences du Vivant), ANR-06-PCVI-0014 BIODENDRIDOT, and CNRS for financial support.