1 Introduction
Metal pollution is one of the major environmental concerns of contemporary society. Many natural waters are polluted by metal ions as a result of their release by industrial plants, chemical manufacturing and mining activities. The presence of heavy metals such as cadmium, lead, copper and zinc in aqueous environments, even in small amounts, may create a major concern because of their toxicity and carcinogenicity to various systems of the human body [1]. Lead and cadmium are deemed the most toxic heavy metals. Their poisoning in humans causes severe damage to the kidney, nervous system, bones and brain [2,3]. Zinc and copper are essential elements for good health, but like all heavy metals, an excess of the metals can be harmful. For example, Zn excess can cause nausea, vomiting and hematemesis [4], while Cu excess can cause Wilson's disease [5]. As heavy metals do not degrade biologically like organic pollutants and can accumulate in living tissues, their presence in drinking water or industrial effluents is a public health problem because of their entry into the food chain through waste discharge into bodies of water. Therefore, more stringent requirements for the removal of heavy metals from wastewaters before release into the environment are required.
Various technologies have been used and developed over the years to remove metal ions, including filtration, chemical precipitation, ion exchange, adsorption and membrane processing. Adsorption is considered one of the most attractive processes for metal ion removal from solution because adsorbents are generally easy to handle, can be regenerated by suitable desorption process, are highly effective, are economical and can be used for various situations without large apparatus [6]. Adsorbents that have been studied for the adsorption of metal ions include activated carbon [7], fly ash [8], peat [9], zeolite [10], manganese oxides [11], kaolinite [12], goethite [13] and resins [14]. However, these adsorbents suffer from low adsorption capacities or removal efficiencies of metal ions. Therefore, researchers have carried out investigations for novel, more promising adsorbents.
Carbon nanotubes (CNTs), a fascinating new member in the carbon family, have attracted special attention after their discovery in 1991 [15] because of their unique morphologies and various potential applications. These tubes consist of rolled graphene sheets, which can be present as single-walled (SWCNTs) or multi-walled (MWCNTs) depending on their preparation conditions. They have been proven to possess great potential as superior adsorbents because they have a large specific surface area and small, hollow, layered structures. CNTs have also been proven to possess great potential for removing many kinds of pollutants, such as ionizable organic compounds from water [16,17], dichlorodiphenyltrichloroethane and its metabolites in water samples [18], organophosphorous pesticides in wastewater sludge [19], chlorophenol [20], atrazine from aqueous solution [21], polyhalogenated organic pollutants in environmental water samples [22–25], aniline [26], pharmaceuticals in spiked water samples [27], drugs in urine [28], virus from water [29], polyaromatic hydrocarbons [30], thiamethoxam, imidacloprid, acetamiprid [31], polycyclic aromatic hydrocarbons in environmental water [32], pesticides [33] and various divalent metal ions from aqueous solution [34–39]. This superior ability of CNTs to bind pollutants comes from strong interaction with the pollutants as a result of the electrons delocalized on the hexagonal arrays of carbon atoms on the surface of CNTs.
The kinetics and mechanism of adsorption of Cd(II), Pb(II), Cu(II) and Zn(II) from aqueous solution by MWCNTs has not yet been well investigated and is not yet well understood [40–43]. In this paper, kinetic studies were performed for the adsorption of cadmium, lead, copper and zinc on pristine MWCNTs in aqueous solution to achieve a better understanding of the adsorption process and to enhance the efficiency of metals ion removal from polluted water.
2 Experimental
2.1 Materials
MWCNTs were obtained from Shenzhen Nanotech Port Co., Ltd were used as received. Analytical grade cadmium, lead, copper and zinc nitrate (Fluka, Ion Standard Solution, 1000 mg/L) were employed to prepare stock solutions each containing 100 mg/L of Cd(II), Pb(II), Cu(II) and Zn(II). The stock solutions were further diluted to the desired ion concentrations. All other chemicals were analytical grade and were obtained from Sigma-Aldrich. The experiments were performed using ultrapure water with resistivity not less than 18.2 MΩcm obtained with a Millipore Milli-Q system (Billerica, USA).
2.2 Characterization techniques
A transmission electron microscope (TEM) (type JEOL JEM-1230 operating at 120 kV attached to a CCD camera) was used to characterize the morphological structure of the MWCNTs. The specific surface area of the different MWCNTs were determined from nitrogen adsorption/desorption isotherms measured at 77 K using a model NOVA 3200e automated gas sorption system (Quantachrome, USA).
2.3 Analytical measurements
The total metal ion concentration was determined by voltammetric measurement using a Metrohm, 797 VA computrace (Switzerland) with a three-electrode system, including hanging mercury as the working electrode, a platinum plate as the counter electrode, and Ag/AgCl (3.0 mol L−1 KCl) as the reference electrode. The metal ion determination was evaluated by differential pulse anodic stripping voltammetry (DPASV).
2.4 Adsorption experiments
Kinetic experiments were carried out to establish the effect of time and temperature on the adsorption process and to identify the adsorption rate. The experimental procedures are described as follows: (1) a series of solutions containing different metal ion concentrations was prepared in 250 mL conical flasks; (2) the pH of the solution was adjusted; (3) the ionic strength was adjusted using a potassium nitrate solution; (4) 125.0 mg of MWCNTs was added into the solution; (5) the solution was stirred on a magnetic stirrer at 400 rpm to homogeneously disperse the MWCNTs in solution for a certain period of time at room temperature, (6) after the completion of preset time intervals, a certain volume of the solution was taken and immediately filtered to collect the supernatant, (7) stirring was then briefly interrupted at predetermined time intervals, and 10.0 mL of supernatant was pipetted from the conical flask and filtered through a 0.45 μm membrane filter. The residual metal ion concentrations in the aqueous solution were then determined by DPASV. Except when the pH effect was studied, all experiments were carried out at an initial pH of 7.0.
The adsorbed metals ions were calculated as follows:
(1) |
The effect of solution pH on metal ion adsorption was studied by dispersing 250.0 mg of MWCNTs into 20.0 mL of a 1 mg/L cadmium, copper, lead or zinc and 0.10 M KNO3 solution. The initial pH values were then adjusted from 3.0 to 6.0 using acetic acid/acetate buffer solution and from 7.0 to 9.0 using universal pH buffer (Britton–Robinson buffer). After the suspensions were stirred for 120.0 minutes at room temperature, they were filtered through 0.45 μm membrane filters. The amounts of metal ions adsorbed on the MWCNTs were calculated as the difference between the initial and the final concentrations at equilibrium.
The MWCNT dose effect on heavy metal ion adsorption was investigated by adding 50.0, 100.0, 150.0, 200.0, 250.0 or 300.0 mg of MWCNTs to a 20.0 mL solution with an initial metal ion concentration of 1 mg/L at a pH value of 7.0. Adsorption of metals ions on the walls of the glass flasks and the filter paper was determined by running a blank experiment without MWCNTs and found to be negligible.
3 Results and discussion
3.1 Characterization of CNTs
Transmission electron microscope imaging was used to study the morphology of the pristine MWCNTs and a representative image is presented in Fig. 1. It is clear from the figure that the pristine MWCNTs are rope-like, curved and highly tangled tubes with diameters between 40 and 60 nm. Because of inter-molecular forces, the pristine MWCNTs of different size and direction form an aggregated structure.
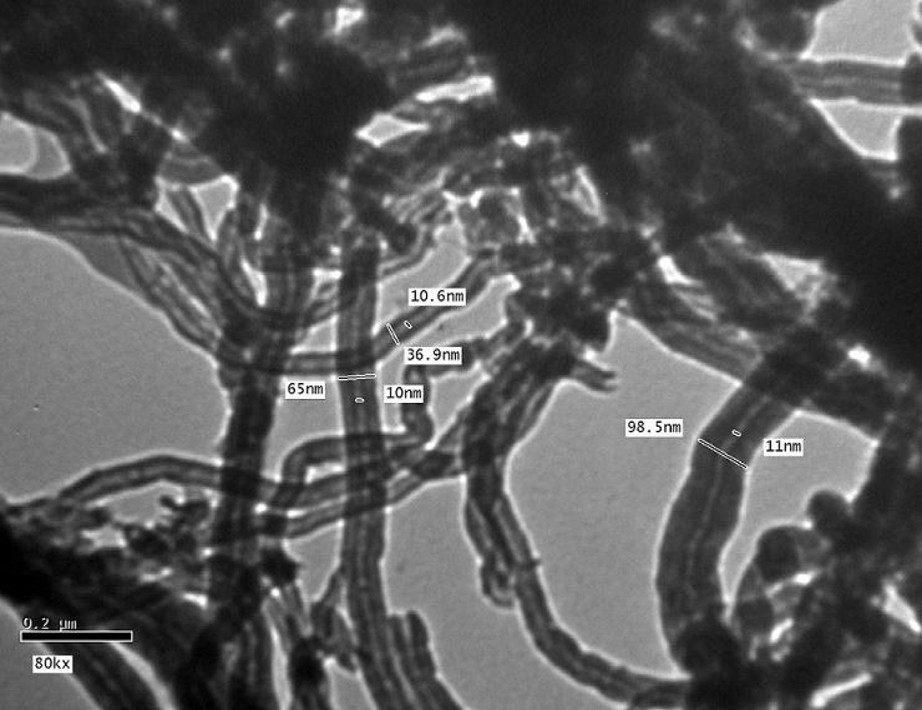
Transmission electron microscope images of pristine MWCNTs.
Nitrogen adsorption/desorption isotherms for the pristine MWCNTs were determined at 77 K, and the results are presented in Fig. 2. According to the original IUPAC classification [44], isotherms obtained with the different CNTs samples are classified as type IV isotherms with H3 type hysteresis loops. However, according to the extended classification of adsorption isotherms [45], the isotherms are classified as type IIb isotherms. The BET specific surface area was found to be 61.5 m2 g−1.
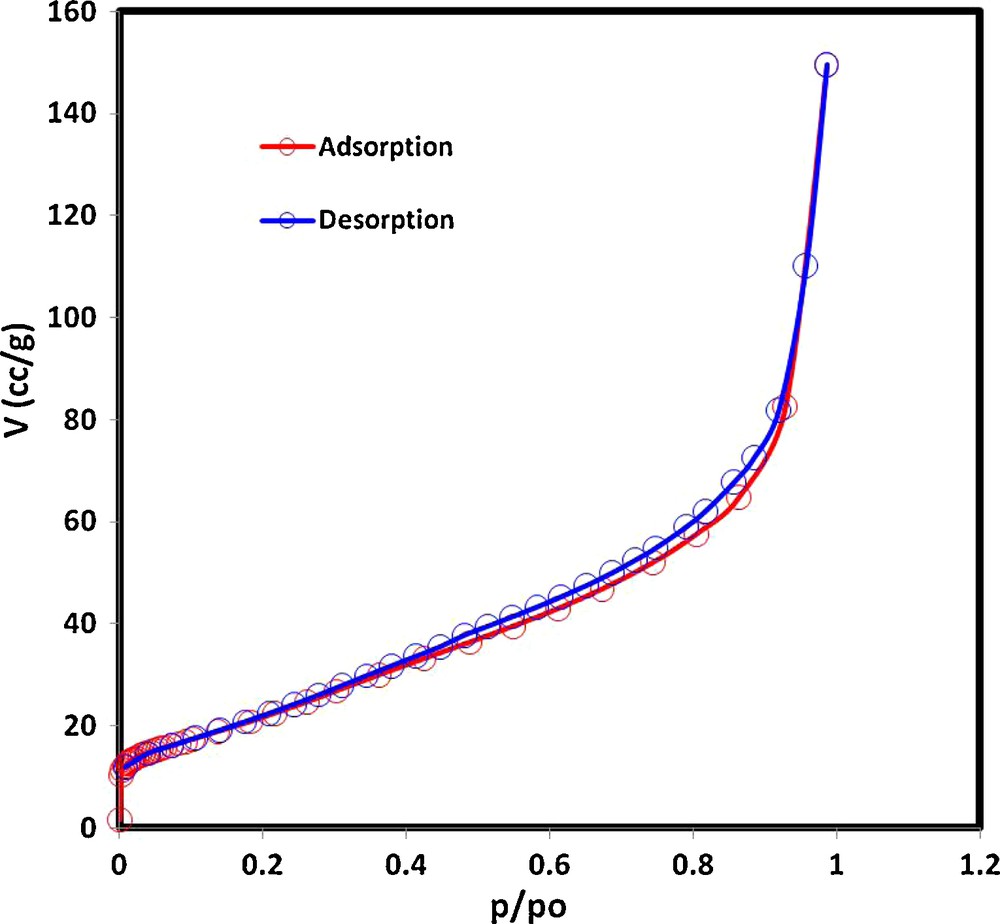
N2 adsorption/desorption isotherms of MWCNTs.
3.2 Adsorption study
Adsorption of metal ions from aqueous solution by carbonaceous adsorbents is controlled by two different interactions. The first interaction is electrostatic, which arises mainly from the surface charges produced on the carbon surface due to immersion in water and the ions present in the solution. The other interaction is non-electrostatic, predominantly van der Waals in nature [46,47]. However, there are many other minor factors that govern both types of interactions and that consequently affect the adsorption process. These factors include the nature of the adsorbent; the solution conditions including pH, ionic strength and temperature; and the nature of the metal ions. Accordingly, the effect of different factors on the interaction, and consequently the adsorption, will be studied.
3.2.1 Effect of contact time
The contact time between the adsorbate and the adsorbent is one-key factor affecting the removal of any pollutants from the environment via adsorption. Fig. 3 show the effect of contact time on the adsorption of Cu(II), Pb(II), Cd(II) and Zn(II) from aqueous solution onto MWCNTs. It is noted that the percentage adsorption of metals ions increased remarkably at the beginning of the experiment and then reached equilibrium. For the four metal ions, the time required to reach equilibrium was very short, approximately 15.0 minutes. According to the results, the equilibrium time was assigned as 120 minutes for the rest of the experiments to be sure that full equilibrium was achieved. The percent adsorbed of metals ions onto MWCNTs reached 84.98%, 31.07%, 13.85% and 81.69% for Cu(II), Pb(II), Cd(II) and Zn(II), respectively, after 120 minutes.

Effect of contact time on the adsorption of Cu(II), Pb(II), Cd(II) and Zn(II) from aqueous solution by MWCNTs (experimental conditions: pH 7; MWCNTs mass, 0.25 g/20 mL; ionic strength, 0.1 M KNO3; metal ion concentration, 1 mg/L).
3.2.2 Effect of MWCNTs mass
The effect of MWCNTs mass on the percentage metal ions adsorbed from aqueous solution was studied at a metal ion concentration of 1 mg/L (Fig. 4). The experimental results revealed that metal ion removal efficiencies increased gradually with increasing amount of MWCNTs used. Specifically, increasing the MWCNTs mass from 0.05 g to 0.25 g sharply enhanced the percentage adsorption of Zn(II) from 47.29 to 72.93%, Cd(II) from 7.77 to 31.44%, Pb(II) from 37.60 to 65.54%, and finally Cu(II) from 48.19 to 85.08%. This may be attributed to the fact that increasing the adsorbent dose provides a greater surface area or more adsorption sites for the metal ions [48]. Further increased in the amount of MWCNTs used from 0.25 g to 0.30 g did not change the percentage of metal ions removed significantly.
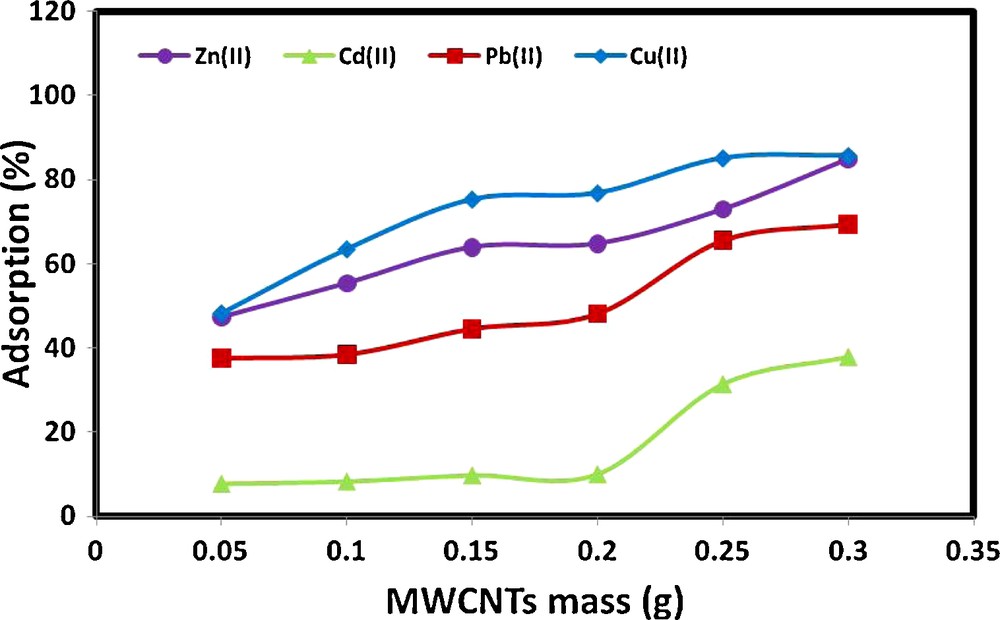
Effect of MWCNTs mass on the adsorption of Cu(II), Pb(II), Cd(II) and Zn(II) from aqueous solution (experimental conditions: pH 7; contact time, 2 hours; ionic strength, 0.1 M KNO3; metal ion concentration, 1 mg/L).
3.2.3 Effect of ionic strength
The influence of the ionic strength on the adsorption of metal ions is great as it creates different adsorption situations in which the electrostatic interactions between the MWCNTs surface and the metal ions are either attractive or repulsive. The ionic strengths of 0.001, 0.01, 0.1 and 1.0 M (KNO3) were chosen to investigate their effect on the Cu(II), Pb(II), Cd(II) and Zn(II) adsorption by MWCNTs. As shown in Fig. 5, the percentage adsorbed metals ions increased with increasing the ionic strength of the solution then it gradually decreased, especially Pb(II), Cd(II) and Zn(II), whereas Cu(II) did not affected that much. This might indicate the attractive nature of the electrostatic interactions between the metal ions and the MWCNTs surface at low ionic strength (i.e., between 0.001 and 0.1 M). Further increasing the ionic strength from 0.1 to 1.0 M KNO3 then changed this interaction to repulsive in nature and hence decreased the adsorption of metal ions.
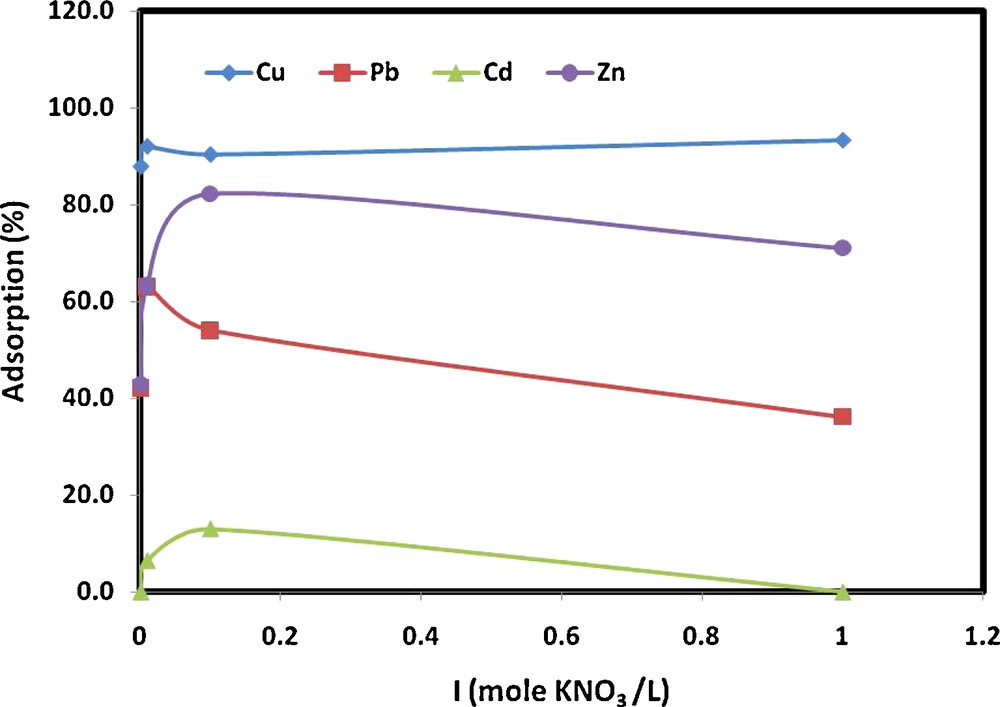
Effect of ionic strength on metal ion adsorption onto MWCNTs (experimental conditions: pH 7; contact time, 2 hours; MWCNT mass, 0.25 g/20 mL; metal ion concentration, 1 mg/L).
Metal ions usually form electric double layer (EDL) complexes with MWCNTs. It has been reported [49] that the presence of a cation, such as K+, decreased heavy metal ion interaction constants because of the accumulation of charge in the vicinity of the MWCNTs surface. This effect created a localized potential that repelled other cations, thus reducing their adsorption potential. In addition, the ionic strength affected the activity coefficients of Pb(II), Cd(II) and Zn(II) ions, which limited their transfer to the MWCNTs surface [50]. Furthermore, an increase in ionic strength supplies more positive ions, which out-compete heavy metals ions for adsorption sites on the MWCNTs.
3.2.4 Effect of pH
The solution pH is one of the dominant parameters controlling the adsorption process, especially for heavy metal ions such as Cu(II), Pb(II), Cd(II) and Zn(II), as they exist in different species depending on the solution pH. The effect of solution pH on the adsorption of Cu(II), Pb(II), Cd(II) and Zn(II) by MWCNTs was therefore explored in the pH range from 2.0 to 9.0 (Fig. 6). In general, the removal of metals ions by MWCNTs was highly dependent on the pH of the solution. For all the metals studied, the percentage adsorption increased gradually with increasing pH. For example, the removal of Cu(II) increased gradually from 12.41 to 90.63%, while from 4.77 to 25.13% for Pb(II), from 0 to 4.23% for Cd(II), and 0.0 to 44.84% for Zn(II), when the pH increased from pH 2.0 to pH 6.0. The minimum adsorption observed at low pH values might be because the higher concentration and mobility of the hydronium ions (H+) present favored the preferential adsorption of hydrogen ions over metal ions. Moreover, at low pH values, the surface of the MWCNTs is mainly covered by H+, which hinders metal ions from approaching the binding sites on the MWCNTs. This finding was also in agreement with the surface complex formation theory (SCF), which states that with an increase in the pH, the competition for the adsorption sites between proton and metal species decreases [51]. Moreover, surface positive charge decreases, which leads to less coulombic repulsion of the metal.
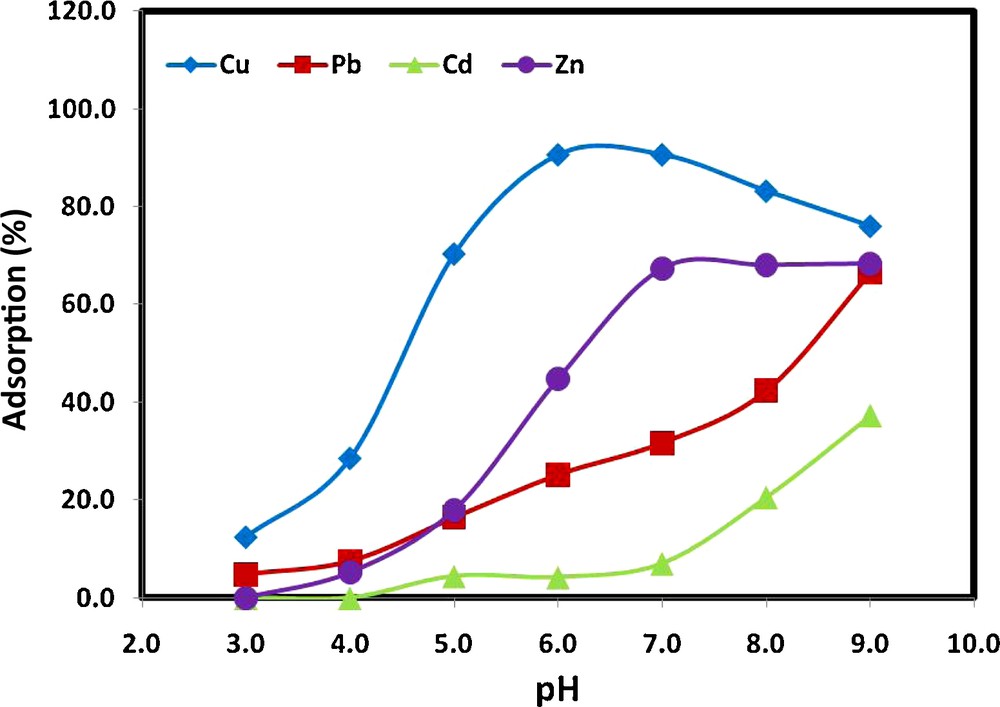
Effect of solution pH on the adsorption of Cu(II), Pb(II), Cd(II) and Zn(II) from aqueous solution by MWCNTs (experimental conditions: contact time, 2 hours; MWCNTs mass, 0.25 g/20 mL; ionic strength, 0.1 M KNO3; metal ion concentration, 1 mg/L).
Further increases in the pH of the solution had different effects on the adsorption process, especially in the basic region where deposition will play the main role in removal. Increasing the pH from 6.0 to 9.0 sharply increased the percentage adsorption of Cd(II) from 4.23 to 37.27%. This increase in the percentage removal was mainly due to adsorption, as cadmium usually precipitates at higher pH values, as is presented in the cadmium speciation diagram [52]. In the case of the lead ions, the percentage adsorption was increased from 25.13 to 66.45% when the pH of the solution increased from 6.0 to 9.0. This increase in the percentage adsorption at a pH higher than 6.0 could be due to the precipitation of lead in the form of Pb(OH)2 [52]. The same effect was observed for copper ions, where the percentage adsorption decreased from 90.63% at pH 6.0 to 76.0% at pH 9.0. This result could again be due to the formation of Cu(OH)2 at pH values higher than 6.0 [52] and competition with cadmium ions present in their free ionic form or with hydroxide ions (HO−), which could cover the MWCNTs surface. In the case of zinc ions, the percentage adsorption increased from 44.84% at pH 6.0 to 68.04% at pH 9.0. This increase could be due to either adsorption at the MWCNTs surface or precipitation of zinc as zincite (ZnO) and/or zinc hydroxides [53]. It is commonly agreed that the adsorption of metal ions, such as copper, lead and zinc, increases with increasing pH as the metal ionic species become less stable in solution. However, at higher pH values (pH 6.0–10.0), there is a decrease in the adsorption capacity. This decrease is due to copper, lead and zinc precipitation.
3.2.5 Effect of metal ion concentration
The effect of metal ion concentration on the adsorption behavior of MWCNTs was studied at a mass of 250 mg, and the results are shown in Fig. 7. Generally, it is clear from the figure that increasing the concentration of metals ions while keeping the amount of the adsorbent constant led to an increase in the percentage of metal ions removed up to 2.5 × 10−5 mol/L. Further increases in the metal ion concentration were accompanied by reduction in the percentage adsorbed. This phenomenon might be due to saturation of the active sites of the MWCNTs with the metal ions at higher concentrations.
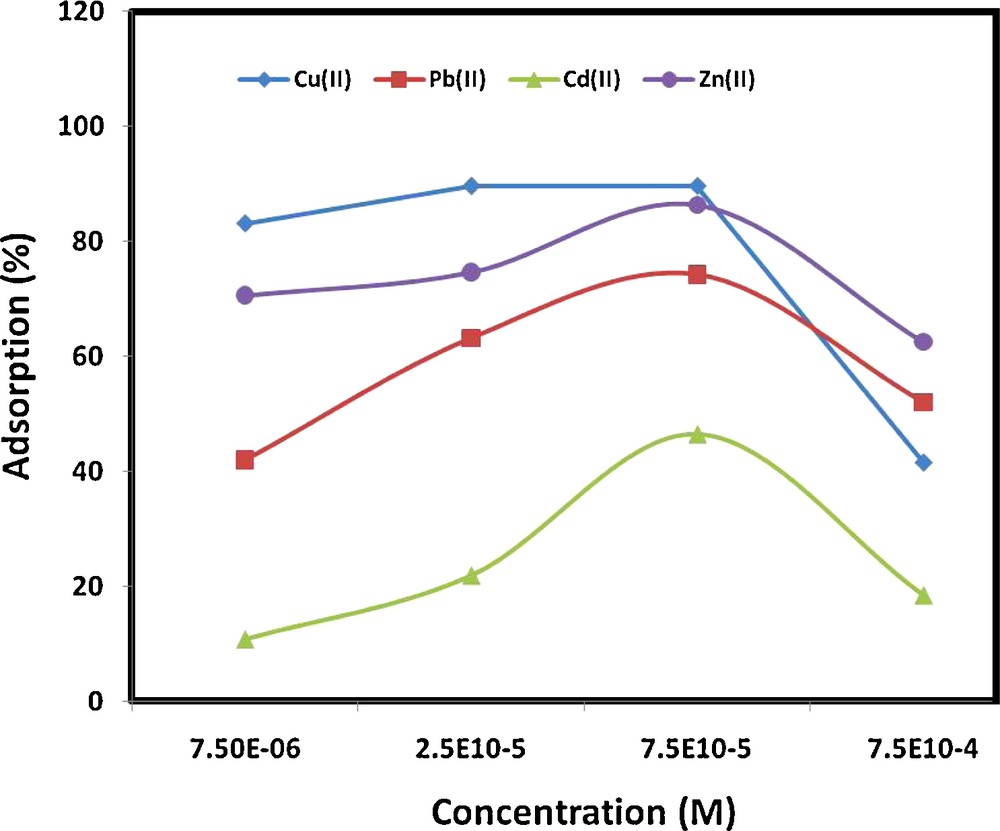
Effect of metal ion concentration on adsorption from aqueous solution (experimental conditions: pH 7; contact time, 2 hours; MWCNTs mass, 0.25 g/20 mL; ionic strength, 0.1 M KNO3).
3.3 Kinetic study
The rates at which metal ions are transferred from the bulk solution to the adsorbent surface and are accumulated there determine the kinetics of adsorption and hence the efficiency of the adsorption process. This study of kinetics thus provides insight into the possible mechanism of adsorption and the reaction pathways. An evaluation of adsorption rate processes also yields valuable information on the interactions and has therefore attracted the interests of almost all involved in experimenting with adsorption on solid surfaces from the liquid phase. A number of theoretical models and equations are available for this purpose, and the best fit of the experimental data to any of these models is interpreted as giving the appropriate kinetics for the adsorption process. The change in the amount of metal ion adsorbed per unit mass of MWCNTs (qt) at any time t was plotted and is presented in Fig. 8. In general, the figure showed that most of the metal ions reached equilibrium within a short period of time. The adsorption kinetics data of metals ions on the adsorbent studied were then analyzed by different kinetic models: the Lagergren pseudo-first order model, the Pseudo-second order model, and the Elovich equation.

Variation in the amount adsorbed of Cu(II), Pb(II), Cd(II) and Zn(II) by MWCNTs with experiment time (experimental conditions: temperature, 298 K; pH 7.0; MWCNTs mass, 0.25 g/20 mL; ionic strength, 0.1 M KNO3).
The Lagergren equation is one of the most common equations describing the rate of adsorption in liquid-phase systems. This equation [54] has been one of the most used equations particularly for pseudo-first order kinetics:
(2) |
The pseudo-second order equation has also been interpreted as a special kind of Langmuir kinetics [55], assuming that: (i) the adsorbate concentration is constant in time; and (ii) the total number of binding sites depends on the amount of adsorbate adsorbed at equilibrium. The linearized form of the pseudo-second order rate equation is given as follows:
(3) |

Pseudo-second order plots for Cu(II), Pb(II), Cd(II) and Zn(II) adsorbed on MWCNTs at different concentrations (experimental conditions: temperature, 298 K; pH 7.0; MWCNTs mass, 0.25 g/20 mL; ionic strength, 0.1 M KNO3).
Table 1 presents the pseudo-second order rate equation parameters calculated from the slope and intercept of the plot of t/qt versus t. It is clear from the data that all the experimental data fit with good correlation coefficient values (R2), which indicated the suitability of the pseudo-second order rate equation for the description of the adsorption of metal ions from aqueous solution by MWCNTs. In addition, the values of the amount of metal ion adsorbed per unit mass of MWCNTs at equilibrium (qe) calculated from the slope of the plot of t/qt versus t were in good agreement with the experimental values. Furthermore, the values of the pseudo-second order rate constant, k2, which often depends on the experimental conditions like initial metal concentration, solution pH and temperature [57,58], decreases with increasing initial adsorbate concentration as a rule. In this scenario, k2 is interpreted as a time-scaling factor. Thus, the higher the initial concentration of adsorbate, the more time is required to reach equilibrium (i.e., the k2 value decreases) [59].
Pseudo-second order parameters for Cu(II), Pb(II), Cd(II) and Zn(II) adsorbed on MWCNTs at different concentrations (experimental conditions: temperature, 298 K; pH 7.0; MWCNTs mass, 0.25 g/20 mL; ionic strength, 0.1 M KNO3).
Concentration (M) | qe (mol/g) | k2 | h | R2 | |
Cu(II) | 2.5 × 10−5 | 1.67 × 10−6 | 5.52 × 105 | 1.54 × 10−6 | 0.999 |
Cu(II) | 1.6 × 10−5 | 1.07 × 10−6 | 5.01 × 105 | 5.71 × 10−7 | 0.998 |
Pb(II) | 2.5 × 10−5 | 8.86 × 10−7 | 1.69 × 105 | 1.32 × 10−7 | 0.993 |
Pb(II) | 4.9 × 10−6 | 1.21 × 10−7 | 2.91 × 106 | 4.29 × 10−8 | 0.998 |
Zn(II) | 2.5 × 10−5 | 1.54 × 10−6 | 3.56 × 105 | 8.44 × 10−7 | 0.999 |
Zn(II) | 1.5 × 10−5 | 1.01 × 10−6 | 1.75 × 106 | 1.77 × 10−6 | 0.999 |
Cd(II) | 2.5 × 10−5 | 4.43 × 10−7 | 1.24 × 106 | 2.44 × 10−7 | 0.999 |
Cd(II) | 8.9 × 10−6 | 1.35 × 10−8 | 8.34 × 105 | 1.52 × 10−8 | 0.978 |
The initial adsorption rate, h, of the pseudo-second order process as t→0 were calculated from the intercept (h = k2qe2), and in general, their values decreased with increasing initial metal ion concentration. The suitability of the pseudo-second order rate equation for the adsorption of metal ions agreed well with literature, but with much lower equilibration times [60–63], which is one of the advantages of CNTs [40,64].
Elovich equation assumes that the actual solid surfaces are energetically heterogeneous and that neither desorption nor interactions between the adsorbed species could substantially affect the kinetics of adsorption at low surface coverage. The linear form of the Elovich equation is given by [65]:
(4) |
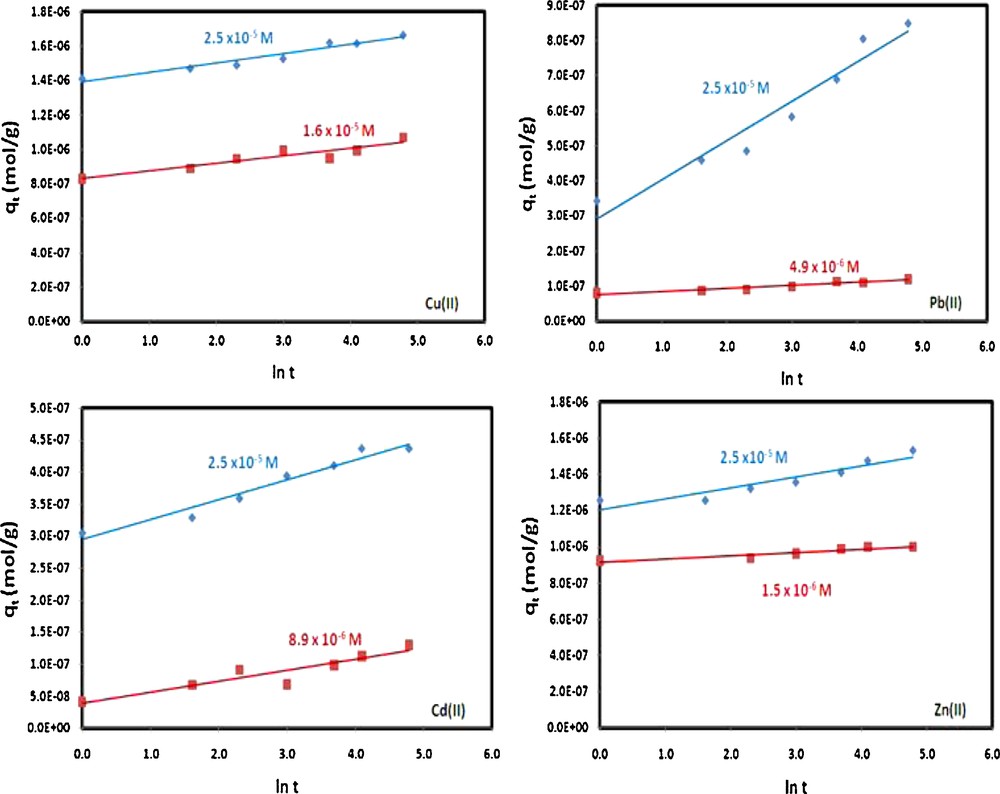
Elovich model plots for Cu(II), Pb(II), Cd(II) and Zn(II) adsorbed on MWCNTs at different concentrations (experimental conditions: temperature, 298 K; pH 7.0; MWCNTs mass, 0.25 g/20 mL; ionic strength, 0.1 M KNO3).
Elovich coefficients for Cu(II), Pb(II), Cd(II) and Zn(II) adsorbed on MWCNTs at different concentrations (experimental conditions: temperature, 298 K; pH 7.0; MWCNT mass, 0.25 g/20 mL; ionic strength, 0.1 M KNO3).
Concentration (M) | α | β | R2 | |
Cu(II) | 2.5 × 10−5 | 2.55 × 1018 | 5.43 × 10−8 | 0.943 |
Cu(II) | 1.6 × 10−5 | 2.70 × 1015 | 4.46 × 10−8 | 0.878 |
Pb(II) | 2.5 × 10−5 | 1.23 × 108 | 1.12 × 10−7 | 0.941 |
Pb(II) | 4.9 × 10−6 | 6.15 × 1011 | 8.81 × 10−9 | 0.919 |
Zn(II) | 2.5 × 10−5 | 7.98 × 1015 | 6.03 × 10−8 | 0.874 |
Zn(II) | 1.5 × 10−5 | 4.41 × 1029 | 1.82 × 10−8 | 0.884 |
Cd(II) | 2.5 × 10−5 | 4.40 × 1011 | 3.10 × 10−8 | 0.956 |
Cd(II) | 8.9 × 10−6 | 5.33 × 108 | 1.74 × 10−8 | 0.866 |
To understand the mechanism by which the adsorption of Cu(II), Pb(II), Cd(II) and Zn(II) from aqueous solution by MWCNTs occurred, two different models were adopted: the intra-particle diffusion model and the liquid film diffusion model. The intra-particle diffusion model is a commonly used technique for identifying the steps involved during adsorption, described by external mass transfer (boundary-layer diffusion) and intra-particle diffusion. The intra-particle diffusion model can be described as [66]:
(5) |
Applying the intra-particle diffusion equation to the experimental data for the adsorption of Cu(II), Pb(II), Cd(II) and Zn(II) from aqueous solution by MWCNTs, straight lines were obtained for all the metal ions under investigation (Fig. 11). The intra-particle diffusion values and the coefficient related to the extent of boundary layer thickness were then calculated from the slope and intercept, and the results are presented in Table 3. Although the correlation coefficients were considerably good, the straight lines did not pass through the origin as expected for an intra-particle diffusion mechanism. The nonzero intercepts of the plots in each case were a clear indication that although intra-particle diffusion is slow, it is not the slowest of the rate processes determining the overall order. The interaction of metal ions with the MWCNTs surface therefore remains the most significant rate process. This phenomenon was also observed in most of the studies discussing the adsorption of metal ions from aqueous solution by different adsorbents in the literature [67–69].
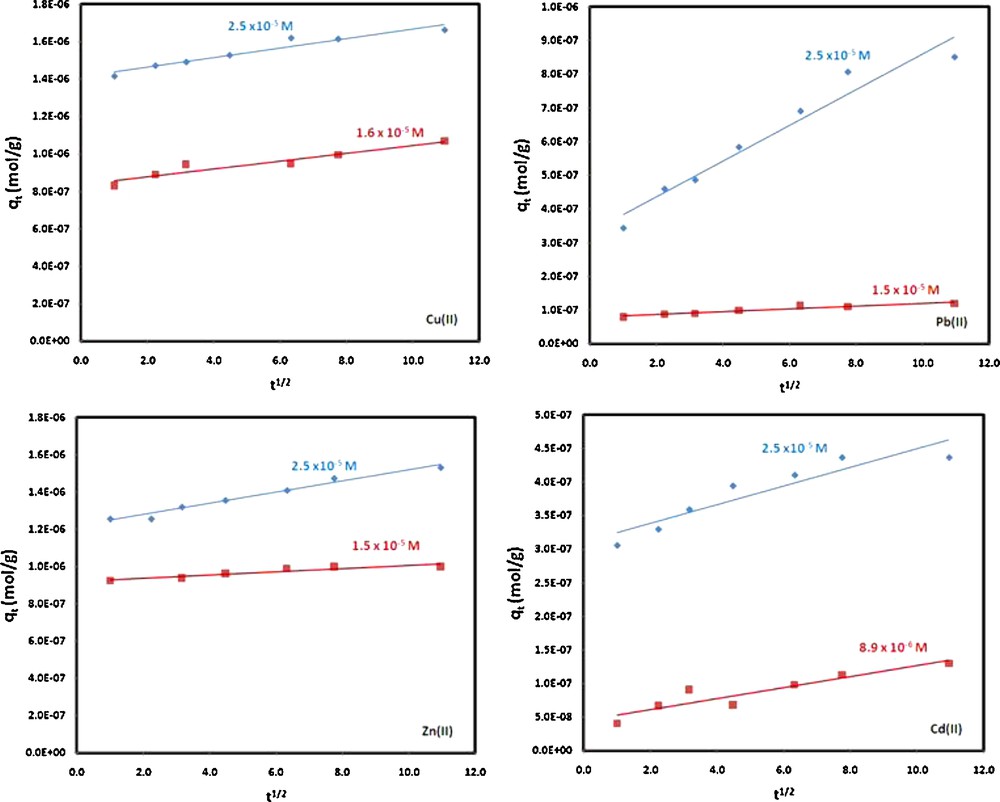
Intra-particle diffusion plots for Cu(II), Pb(II), Cd(II) and Zn(II) adsorbed on MWCNTs at different concentrations (experimental conditions: temperature, 298 K; pH 7.0; MWCNT mass, 0.25 g/20 mL; ionic strength, 0.1 M KNO3).
Intra-particle diffusion coefficients for Cu(II), Pb(II), Cd(II) and Zn(II) adsorbed on MWCNTs at different concentrations (experimental conditions: temperature, 298 K; pH 7.0; MWCNTs mass, 0.25 g/20 mL; ionic strength, 0.1 M KNO3).
Concentration (M) | Kid (mol/g) | C | R2 | |
Cu(II) | 2.5 × 10−5 | 2.54 × 10−8 | 1.41 × 10−6 | 0.929 |
Cu(II) | 1.6 × 10−5 | 2.08 × 10−8 | 8.38 × 10−7 | 0.911 |
Pb(II) | 2.5 × 10−5 | 5.29 × 10−8 | 3.32 × 10−9 | 0.950 |
Pb(II) | 4.9 × 10−6 | 4.15 × 10−9 | 7.90 × 10−8 | 0.919 |
Zn(II) | 2.5 × 10−5 | 3.00 × 10−8 | 1.22 × 10−6 | 0.974 |
Zn(II) | 1.5 × 10−5 | 8.59 × 10−9 | 9.21 × 10−7 | 0.857 |
Cd(II) | 2.5 × 10−5 | 1.39 × 10−8 | 3.11 × 10−7 | 0.866 |
Cd(II) | 8.9 × 10−6 | 8.14 × 10−9 | 4.53 × 10−8 | 0.856 |
Liquid film diffusion is a model applied when the flow of the adsorbate through the liquid film surrounding the adsorbent particles is the slowest process determining the kinetics of the rate. The liquid film diffusion model is given by the following equation:
(6) |
3.4 Competition among metal ions
Competitive adsorption among metal ions is important in aqueous solution and wastewater treatment because metal ions naturally exist together. Given the above results, it is noteworthy to discuss the competition among the four metal ions under investigation (Cu(II), Pb(II), Cd(II) and Zn(II)) for binding of the active sites present on the MWCNTs surface. It was subsequently observed that binding followed the order Cu(II) > Zn(II) > Pb(II) > Cd(II). Thus, even though all those metals are bivalent, differences in the capacity and thus interactions with the adsorption centers exist.
Unfortunately, there is no consensus between researchers regarding the competitive adsorption of metal ions as researchers have attributed the different affinities to different factors. These factors must be related to the properties of these ions in aqueous solution, which may affect the energy of surface binding and interactions or the accessibility of centers, which can be linked to the sizes of the species to be adsorbed. Although Pb(II) and Cd(II) have the largest radii (1.33 Å and 0.97 Å, respectively), they showed lower adsorption than Cu(II) and Zn(II), which have smaller ionic radii (0.72 Å and 0.74 Å, respectively). Thus, the smaller the ionic radius, the easier it is for a metal ion to penetrate through the boundary layer and adsorb on the MWCNTs surface. Electronegativity (Pauling) was in the following order: Pb(II) > Cu(II) > Cd(II) > Zn(II) (2.33, 1.90, 1.69, and 1.65, respectively). This order did not agreed well with the experimental affinities for binding and adsorption by MWCNTs. The high stability of Cu(II) to adsorption and binding to the MWCNTs compared with other metals can be explained. Since Cu(II) is predominantly specifically adsorbed (inner-sphere complexation), it can be expected that increasing the amount of more strongly bonded Cu reduces the number of sites available for Cd and Zn adsorption. Furthermore, Cu(II) is stabilized by the Jahn-Teller effect. The d9 electronic configuration of this ion gives three electrons in two degenerate eg orbitals and six electrons in the t2g, leading to a doubly-degenerate electronic ground state and a large energetic stabilization. A similar effect was observed in a multi-component system where an increase in the Cu concentration resulted in a reduction in the uptake of other heavy metals [70]. Additionally, it was reported that high copper adsorption was caused by its ability to be reduced by the carbonaceous surface. Therefore, Cu(II) in close proximity of the MWCNTs surface is reduced after being attracted to cation exchange centers on the nanotubes surface and then vacates the cation exchange site for the adsorption of another ion[41].
The lower adsorption of Cd(II) might be because of its lower tendency to form hydrolysis products and that its ions do not compete effectively for variable charge surfaces, such as MWCNTs. As a result, its adsorption is more restricted to permanent charge sites [71]. The difference in the adsorption affinity in this study compared with other studies could be attributed to the unique features of each of the CNTs as the production methods were vastly different.
4 Conclusions
MWCNTs were capable of removing Cu(II), Pb(II), Cd(II) and Zn(II) from aqueous solution. Transmittance electron microscope images showed the MWCNTs as entangled and long nanotubes with average diameters between 40 and 60 nm. Nitrogen adsorption/desorption isotherms at 77 K revealed that the BET specific surface area was 61.524 m2 g−1. In addition, the parameters affecting the adsorption process were optimized, and the adsorption of Cu(II), Pb(II), Cd(II) and Zn(II) was found to generally increase with increasing solution pH and MWCNTs mass, while decrease with increasing solution ionic strength. Many adsorption kinetics models were tested, but the adsorption of the metal ions by MWCNTs was in better agreement with pseudo-second order kinetics and the Elovich model. An intra-particle diffusion mechanism was subsequently adopted, but the results showed that intra-particle diffusion was not the slowest of the rate processes determining the overall order. The interaction of metal ions with the MWCNTs surface likely remains the most significant rate process. Moreover, there was a competition among the metal ions for the active sites present on the MWCNTs surface with an affinity order of Cu(II) > Zn(II) > Pb(II) > Cd(II).