1 Introduction
Tetranuclear complexes involving nickel ions and diketones or diketone-equivalent ligands such as salicylaldehyde have been known for a long time [1–5]. The vertexes of the cubane structure are alternately occupied by four Ni atoms and four methoxo groups, so that each Ni ion is surrounded by three μ3-methoxo groups. There are also examples in which the μ3-methoxo groups have been replaced by μ-N3 azido bridges [6,7]. In a large majority of cases ferromagnetic Ni-Ni interactions are active in these nickel cubane complexes, thus giving a S = 4 ground state. Furthermore, magnetic anisotropy can also be present. The growing interest in single-molecule magnets (SMM), molecules that require high-spin ground state and appreciable negative magnetic anisotropy, has thus given a new interest to these cubane complexes [8–12]. Being interested in the syntheses of 3d-4f complexes, we have shown that the o-vanillin ligand is able to link 3d ions through its phenoxo and aldehyde oxygen atoms when 4f ions prefer coordination through the phenoxo and methoxy oxygen atoms [13]. We [14] and other workers [15] have also shown that free methoxy groups can be involved in hydrogen bonds in presence of hydrogen-donor molecules. Recently, the structural determinations of nickel cubane complexes derived from 2-hydroxy-3-methoxy-benzaldehyde (o-vanillin) [16–18] or 2-hydroxy-3-ethoxy-benzaldehyde [19] appeared in the literature. Surprisingly, they were prepared according to a reaction process resulting from hydrolysis of a Schiff base ligand in presence of potassium hydroxide [16] or by reaction of the o-vanillin or equivalent ligands in presence of nickel salts, chloride or perchlorate, and different bases used as deprotonating agents [17–19]. In the present work, we want to describe a more direct synthesis of the nickel cubane complex, starting with o-vanillin and nickel acetate, along with the structural determination and a complete study of the isolated Ni4 complex. Then two chemical ways that allow a self-assembling of these Ni4 units by direct coordination bonds or by introduction of a hydrogen-bond network will be described.
2 Experimental
2.1 Materials
The metal salts, Ni(CH3COO)2·4H2O, Ni(NO3)2·6H2O, Dy(NO3)3·6H2O, and o-vanillin (2-hydroxy-3-methoxy-benzaldehyde), L-valine, 1,3-propanediol, 2-methoxyethylylamine, 3-methoxypropylamine, triethylamine (Aldrich) were used as purchased. High-grade solvents were used for preparing the complexes.
2.2 Complexes
[(MeOH)Ni(OMe)(ovan)]4 (1). (A) o-vanillin (0.15 g, 1 mmol), L-valine (0.12 g, 1 mmol) and Ni(NO3)2·6H2O (0.29 g, 1 mmol) were dissolved with stirring in methanol (30 mL) at room temperature. A methanol solution (10 mL) of Dy(NO3)3·6H2O (0.45 g, 1 mmol), followed by 0.5 mL of triethylamine were added. The intense green solution was filtered 1 hour later. Slow evaporation yielded green crystals 2 days later. Yield: 0.09 g (33%). Anal. Calcd for C40H56Ni4O20 (1091.6): C, 44.0; H, 5.2. Found: C, 43.8; H, 5.0. IR (ATR): 3251l, 2928w, 2822w, 1625s, 1606m, 1538m, 1468m, 1434m, 1417m, 1336m, 1239m, 1201s, 1169w, 1073w, 1054m, 1037 m, 961m, 857w, 746m, 725m, 643w cm−1.
(B) o-vanillin (1.5 g, 10 mmol) and Ni(CH3COO)2·4H2O (2.5 g, 10 mmol) were dissolved with stirring in methanol (60 mL) at room temperature. Then 2 mL of triethylamine were added and the mixture was heated to 50 °C for 40 min. The cooled mixture was filtered, yielding a green precipitate that was washed with diethyl ether. Yield: 2.3 g (83%). Slow diffusion of diethyl ether to a methanol solution of the green precipitate gave green crystals suitable for XRD analysis. Anal. Calcd for C40H56Ni4O20 (1091.6): C, 44.0; H, 5.2. Found: C, 43.9; H, 5.1. IR (ATR): 3251l, 2928w, 2822w, 1625s, 1606m, 1538m, 1468m, 1434m, 1417m, 1336m, 1239m, 1201s, 1169w, 1073w, 1054m, 1037m, 961m, 857w, 746m, 725m, 643w cm−1.
(HOCH2CH2CH2OH)2Ni4(OMe)4(ovan)4 (2). Complex 1 (0.2 g, 0.18 mmol) was partially dissolved in acetone (10 mL) and 1,3-propanediol (0.2 g, 2.6 mmol) was added. The resulting mixture was stirred and heated to 40 °C for 30 min, then filtered and left to evaporate. A green powder was filtered off 3 h later, washed with diethyl ether and dried. Yield: 0.13 g (65%). Anal. Calcd for C42H56Ni4O20 (1115.6): C, 45.2; H, 5.1. Found: C, 45.3; H, 5.1. IR (ATR): 3493l, 3146l, 2937w, 2857w, 1619s, 1541m, 1467m, 1439m, 1413m, 1338m, 1240m, 1203s, 1168w, 1072w, 1051m, 963m, 859w, 723m, 644w cm−1.
(CH3OCH2CH2NH2)4Ni4(OMe)4(ovan)4 (3). Complex 1 (0.2 g, 0.18 mmol) was partially dissolved in acetone (10 mL) and 2-methoxyethylylamine (0.2 g, 2.7 mmol) was added. The resulting mixture was stirred for 2 hours, yielding a green precipitate that was filtered off, washed with diethyl ether and dried. Yield: 0.13 g (54%). Anal. Calcd for C48H76N4Ni4O20 (1263.9): C, 45.6; H, 6.1; N, 4.4. Found: C, 45.3; H, 6.2; N, 4.2. IR (ATR): 3332w, 3241w, 3163m, 2942w, 2876m, 2800m, 1622s, 1604m, 1536m, 1470m, 1435m, 1416m, 1342m, 1234m, 1200s, 1145w, 1122w, 1073w, 1055m, 959m, 855w, 746m, 722 m, 641w cm−1.
(CH3OCH2CH2CH2NH2)4Ni4(OMe)4(ovan)4 (4). The same experimental procedure and use of an excess of 3-methoxypropylamine (0.2 g, 2.2 mmol) instead of 2-methoxyethylylamine yielded a green precipitate that was filtered off, washed with diethyl ether and dried. Yield: 0.13 g (55%). Anal. Calcd for C52H84N4Ni4O20 (1320.0): C, 47.3; H, 6.4; N, 4.2. Found: C, 47.0; H, 6.2; N, 4.1. IR (ATR): 3330w, 3243w, 3162m, 2928w, 2880m, 2803m, 1625s, 1604m, 1536m, 1472m, 1438m, 1410m, 1343m, 1235m, 1200s, 1165w, 1112m, 1072w, 1054m, 1016m, 958m, 856w, 744w, 734w, 722m, 640w cm−1.
(HOCH2CH2NH2)4Ni4(OMe)4(ovan)4 (5). The same experimental procedure and use of an excess of ethanolamine (0.2 g, 3.3 mmol) yielded a green precipitate that was filtered off, washed with diethyl ether and dried. Yield: 0.18 g (82%). Anal. Calcd for C44H68N4Ni4O20 (1207.8): C, 43.8; H, 5.7; N, 4.6. Found: C, 43.5; H, 5.5; N, 4.4. IR (ATR): 3465l, 3336w, 3254w, 3174w, 2939w, 2881w, 2808w, 1626s, 1606m, 1538m, 1469m, 1436m, 1419w, 1345m, 1237m, 1203s, 1167w, 1061m, 1030w, 959m, 856w, 747m, 744m, 642w cm−1.
2.3 Materials and methods
All starting materials were purchased from Aldrich and were used without further purification. Elemental analyses were carried out by the Service de microanalyse du laboratoire de chimie de coordination, Toulouse (C, H, N). Magnetic data were obtained with a Quantum Design MPMS SQUID susceptometer. Magnetic susceptibility measurements were performed in the 2–300 K temperature range in a 0.1 T or 1 T applied magnetic field, and diamagnetic corrections were applied by using Pascal's constants [20]. Isothermal magnetization measurements were performed up to 5 T at 2 K. The magnetic susceptibilities have been computed by exact calculations of the energy levels associated to the spin Hamiltonian through diagonalization of the full matrix with a general program for axial symmetry [21], and with the MAGPACK program package [22] in the case of magnetization. Least-squares fittings were accomplished with an adapted version of the function-minimization program MINUIT [23].
2.3.1 Crystallographic data collection and structure determination for (1)
Crystals of 1 were kept in the mother liquor until they were dipped into oil. The chosen crystals were mounted on a Mitegen micromount and quickly cooled to 180 K. The selected crystal of 1 (green, 0.25 × 0.15 × 0.05 mm3) was mounted on an Oxford-Diffraction XCALIBUR (4) using a graphite monochromator (λ = 0.71073 Å) and equipped with an Oxford Cryosystems cooler device. The data were collected at 180 K. The unit cell determination and data integration were carried out using the CrysAlis RED package for the data recorded on the Xcalibur [24]. Seventeen thousand three hundred and ninety-five reflections were collected for 1, of which 2420 were independent (Rint = 0.0302). The structure has been solved by Direct Methods using SIR92 [25], and refined by means of least-squares procedures on a F2 with the aid of the program SHELXL97 [26] included in the software package WinGX version 1.63 [27]. The Atomic Scattering Factors were taken from International tables for X-Ray Crystallography [28]. All hydrogen atoms were geometrically placed and refined by using a riding model. All non-hydrogen atoms were anisotropically refined, and in the last cycles of refinement a weighting scheme was used, where weights are calculated from the following formula: where . Drawings of molecules are performed with the program ORTEP32 [29] with 30% probability displacement ellipsoids for non-hydrogen atoms. CCDC 863778 contains the supplementary crystallographic data for this paper. These data can be obtained free of charge at www.ccdc.cam.ac.uk/conts/retrieving.html or from Cambridge Crystallographic Data Centre, 12, Union Road, Cambridge CB2 1EZ, UK [fax: Internat +44 1223/336 033; deposit@ccdc.cam.ac.uk].
3 Results and discussion
The reaction of o-vanillin with nickel acetate in ethanol and a 2/1 ratio yields the well-known Ni(ovan)2·2H2O complex. In order to find a synthetic route to the cubane complex 1, which was first prepared by pure serendipity, we made several trials. Indeed, heating with stirring a methanol solution of o-vanillin with nickel acetate in a 1/1 ratio and in presence of triethylamine, gives a green precipitate that corresponds to the expected cubane complex in correct yield. Analytical data and structural determination do confirm its [(MeOH)Ni(OMe)(ovan)]4 formulation. This cubane possesses a methanol molecule in the nickel coordination sphere, as cubanes resulting from bidentate ligands. Working in acetone solution, we tried to replace this coordinated methanol molecule by a diol such as 1,3-propanediol. Although we could not obtain crystals, analytical data for the isolated powder complexes are in agreement with a [(HOCH2CH2CH2OH)2Ni4(OMe)4(ovan)4]n formulation. Presence of only two diol molecules in the complex corresponds to an assembling of the cubane units through diol bridges. On the contrary, the [(MeOH)Ni(OMe)(ovan)]4 complex does not react with pyridine or a primary amine ligand. And reaction with an amino-alcohol or an amino-ether ligand yields new compounds in which each methanol molecule is replaced by the reacting amino-alcohol (ethanolamine) or an amino-ether (2-methoxyethylylamine or 3-methoxypropylamine) ligand. From these experimental results, it becomes clear that the oxygen atom of the amino-alcohol or an amino-ether ligand is linked to the nickel ion. A confirmation is given by visible spectra of the complexes 3 and 4 that give absorption bands at 590 nm, similar to the one of the starting [(MeOH)Ni(OMe)(ovan)]4 entity (600 nm), in agreement with a O6 environment of the nickel ions.
3.1 Structural study of [(MeOH)Ni(OMe)(ovan)]4 (1)
The crystallographic data of complex 1 are summarized in Table 1 while a view of the structure is reported in Fig. 1. Relevant bond distances and angles are collated in the figure caption. The structure crystallizes in the tetragonal I41/a space group. The vertexes of the cubane structure are alternately occupied by four Ni atoms and four methoxo groups, so that each Ni ion is surrounded by three μ3-methoxo groups. The octahedral Nickel coordination sphere is completed by two oxygen atoms coming from an o-vanillin ligand (phenoxo and aldehyde oxygen atoms) and by the oxygen atom of a methanol molecule. The Ni-O bond lengths of the chelating ovan ligand are slightly shorter (2.000(1) and 2.027(1) Å) than the three Ni-O(OMe) bonds (2.035(1), 2.039(1) and 2.058(1) Å) of the μ3-bridging methoxo groups, the Ni-O(methanol) bond being slightly longer, 2.097(1) Å. The Ni-O-Ni angles involving the bridging OMe ligands are very similar, 96.41(5), 97.17(5) and 97.87(5)° so that the intramolecular Ni…Ni distances are homogeneous, 3.0332(3), 3.0332(3) and 3.0861(3) Å. The OH groups of the methanol molecules coordinated to the nickel ions act as donors for the phenoxo and methoxy oxygen atoms of the nearest o-vanillin ligand, thus establishing intramolecular bifurcated hydrogen-bond interactions [17]. The Ni ions belonging to two different cubanes are separated by 9.540(4) Å.
Crystallographic data for [(MeOH)Ni(OMe)(ovan)]4 1.
1 | |
Chemical formula | C40H56Ni4O20 |
Formula weight | 1091.61 |
Space group | I 41/a (No. 88) |
a, Å | 22.2370 (4) |
b, Å | 22.2370 (4) |
c, Å | 9.5830 (3) |
α, deg | 90 |
β, deg | 90 |
δ, deg | 90 |
V, Å3 | 4738.64 (19) |
Z | 4 |
ρcalcd, g cm−3 | 1.530 |
λ, Å | 0.71073 |
T, K | 180 |
Gof | 1.046 |
μMoKα, mm−1 | 1.640 |
Ra obs, all | 0.0231, 0.0317 |
wRbobs, all | 0.0577, 0.0596 |
Δρmax, Δρmin, eÅ−3 | 0.294−0.411 |
a R = Σ||Fo|−|Fc||/Σ|Fo|.
b wR = [Σw(|Fo2|−|Fc2|)2/Σw|Fo2|2]1/2.
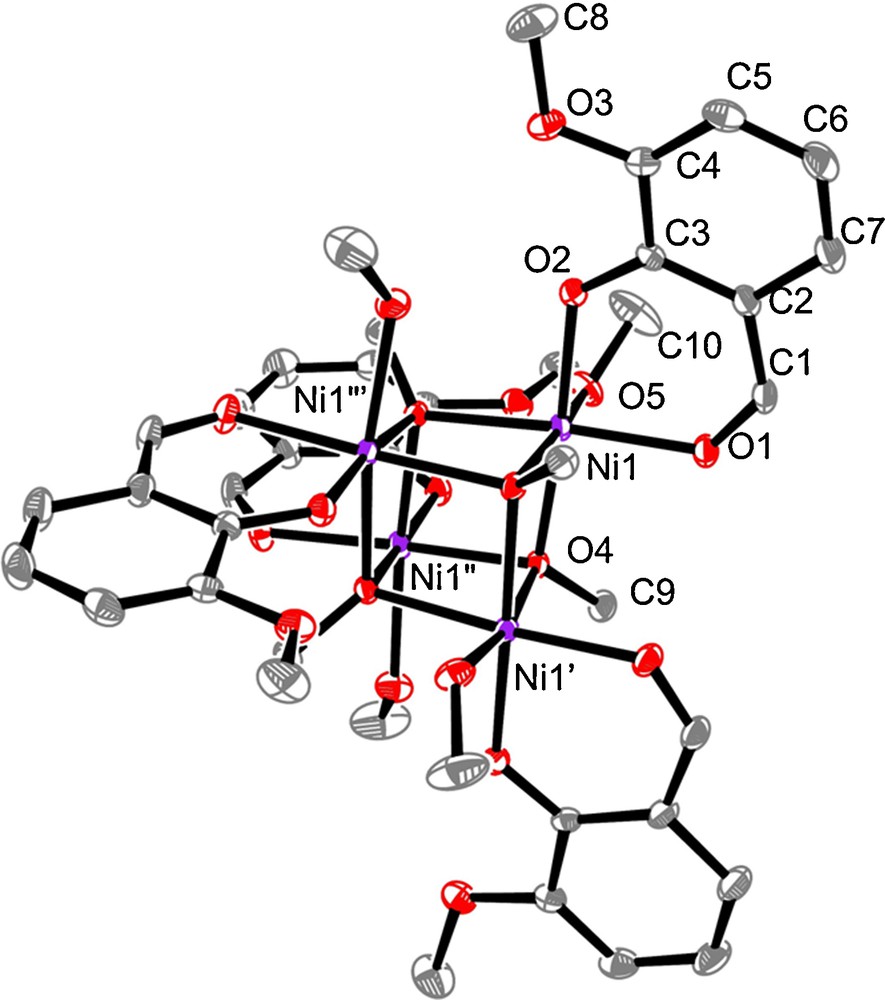
Plot of complex 1 with ellipsoids drawn at the 30% probability level and with partial atom numbering. H atoms are omitted for clarity. Selected bond lengths [Å] and angles [deg]: Ni(1)-O(1) 2.026(1), Ni(1)-O(2) 2.000(1), Ni(1)-O(4) 2.035(1), Ni(1)-O(4′) 2.039(1), Ni(1)-O(4′′) 2.058(1), Ni(1)-O(1) 2.097(1), Ni(1)-O(4)-Ni(1′), 97.17(5), Ni(1)-O(4)-Ni(1′′), 97.87(5), Ni(1′)-O(4)-Ni(1′′) 96.41(5). Symmetry operations: ′: y−1/4, −x + 1/4, −z + 1/4; ′′: −x, −y + 1/2, z; #3 −y + 1/4, x + 1/4, −z + 1/4.
3.2 Magnetic properties
We report in Fig. 2 the magnetic behavior of complex 1 in the form of the thermal variation of the χMT product (χM is the molar magnetic susceptibility corrected for the diamagnetism of the ligands) [20]. In the present case, the χMT product, which is equal to 6.2 cm3 mol−1 K at 300 K, increases slowly to 100 K (7.5 cm3 mol−1 K), then more abruptly to 11 K where it passes by a maximum (12.2 cm3 mol−1 K) before decreasing to 9.1 cm3 mol−1 K at 2 K. The χMT at room temperature is larger than expected for four isolated NiII ions with g = 2 but we must remember that g is larger than two for nickel ions. The experimental data indicate the occurrence of an overall ferromagnetic interaction in complex 1. Finally fitting the experimental data to a simple model derived from the spin only Hamiltonian for a tetranuclear (Ni4) complex, H = -2 JNiNi (SNi1.SNi2 + SNi1.SNi3 + SNi1.SNi4 + SNi2.SNi3 + SNi2.SNi4 + SNi3.SNi4) yields the following data, JNiNi = 5.8 cm−1, g = 2.26 with a R factor equal to 4.0 × 10−4, R = Σ[(χMT)obs − (χMT)calc]2/Σ[(χMT)obs]2. In order to fit the χMT decrease below 12 K, we first introduced a common molecular-field parameter zJ that was found equal to −0.027 cm−1. As we are dealing with a tetranuclear nickel complex, the single-ion anisotropy of nickel ions induces presence of zero-field splitting terms (ZFS) that can be responsible for the χMT decrease at low temperature. The measured value of magnetization at 5 T and 2 K temperature (Fig. 3) indicates that we have a S = 4 ground state, M = 8.5 Nβ against the expected value of 8 for g = 2. With use of the MAGPACK program, the best simulation reported in Fig. 3 gives JNiNi = 5.9 cm−1, g = 2.26, DNi = 2.0 cm−1, along with a low rhombic term E, ENi = 0.4 cm−1.
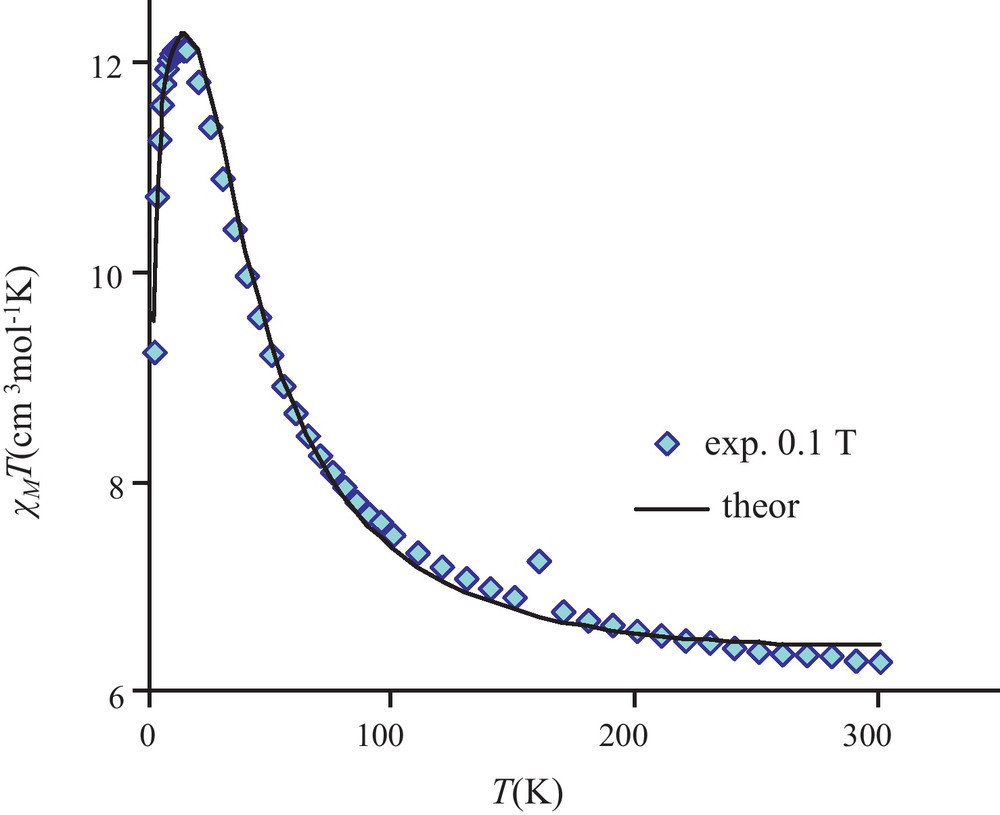
Temperature dependence of the χMT product for complex 1. Solid line corresponds to the best data fit (see the text).
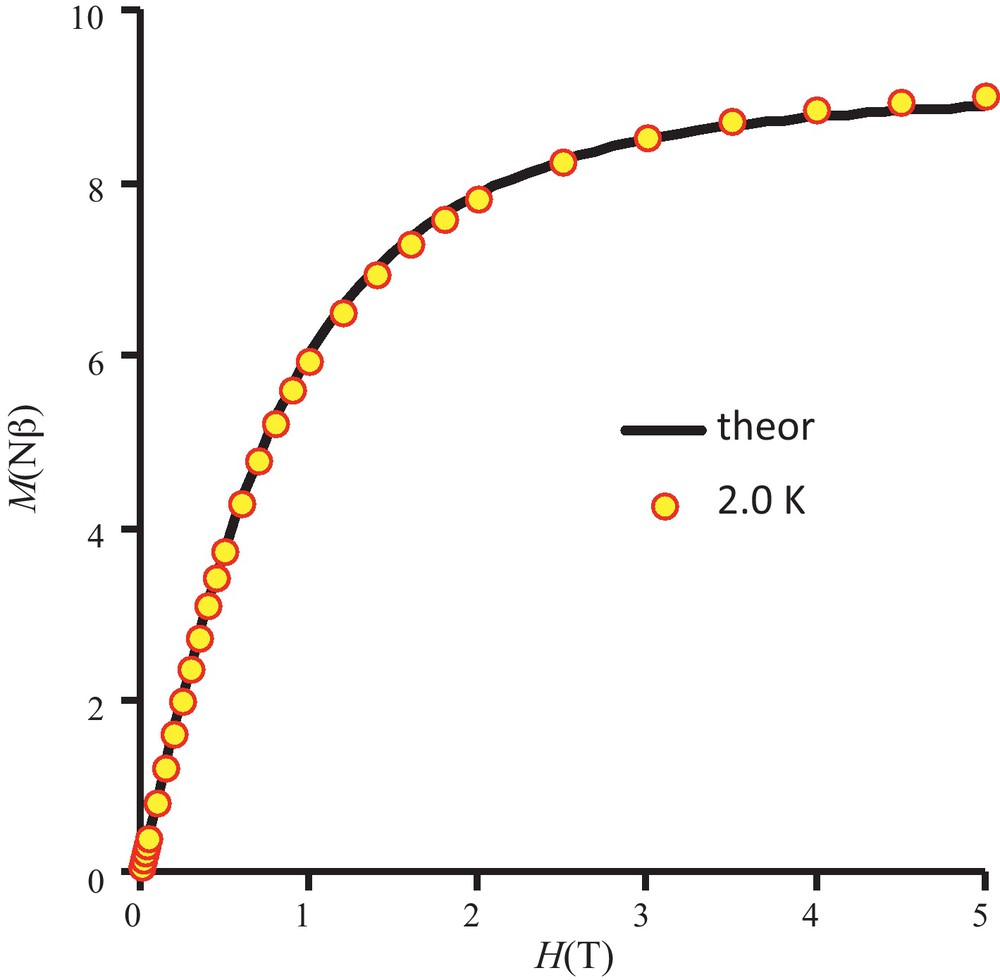
Field dependence of magnetization for complex 1. Solid line corresponds to the best data fit (see the text).
The temperature dependence of the magnetic susceptibility for complex 3 in the 2–300 K temperature range and at different fields is shown in Fig. 4 in the χMT vs. T form. χMT is equal to 4.9 cm3 mol−1 K at 300 K and remains practically constant to 100 K (5.5 cm3 mol−1 K) under an applied 0.1 T field. The χMT product increases first gradually to 30 K (8.5 cm3 mol−1 K) and then sharply below 30 K to a maximum value of 24.2 cm3 mol−1 K at 14 K before decreasing to 7.2 cm3 mol−1 K at 2 K. This sharp χMT increase suggests the onset of a ferromagnetic order. Measurements at different and higher magnetic fields confirm that the magnetic ordering is suppressed with an applied field equal or higher than 1.0 T (Fig. 4 and Fig. S1). The magnetic susceptibility data registered under a 1.0 T field have been computed by the exact calculation of the energy levels through diagonalization of the full energy matrix with a Hamiltonian similar to the one described above but in which an axial ZFS term for NiII ions is introduced. The best fit (solid line, Fig. 5) yields the following data: JNiNi = 3.9 cm−1, g = 2.11, DNi = 7.6 cm−1 with R = 7 × 10−4. In order to check the validity of these results, MAGPACK simulation of magnetization data at 2 K with the above parameters confirms that a lower DNi value (4.0 cm−1), associated to a low ENi term (0.3 cm−1), appears more appropriate (Fig. 6).
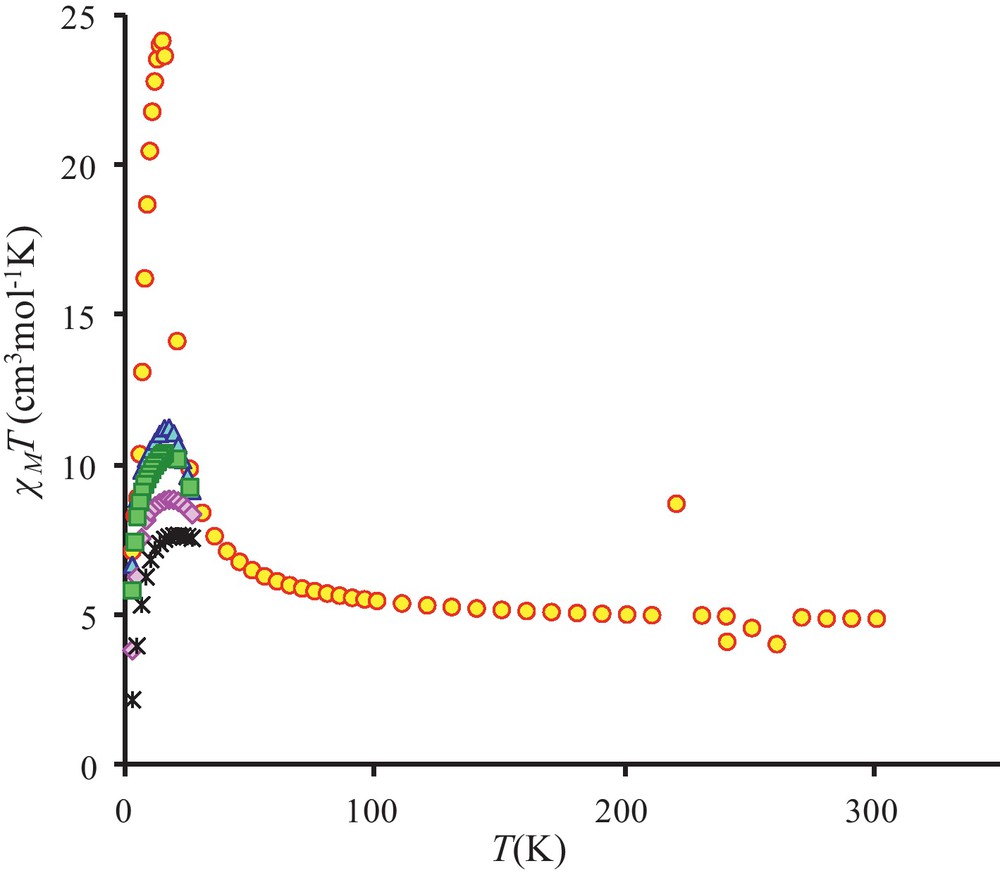
Temperature dependence of the χMT product for complex 3 at different applied magnetic fields: 0.1 T (circles), 0.7 T (triangles), 1.0 T (squares), 2.0 T (diamonds) and 5.0 T (stars).
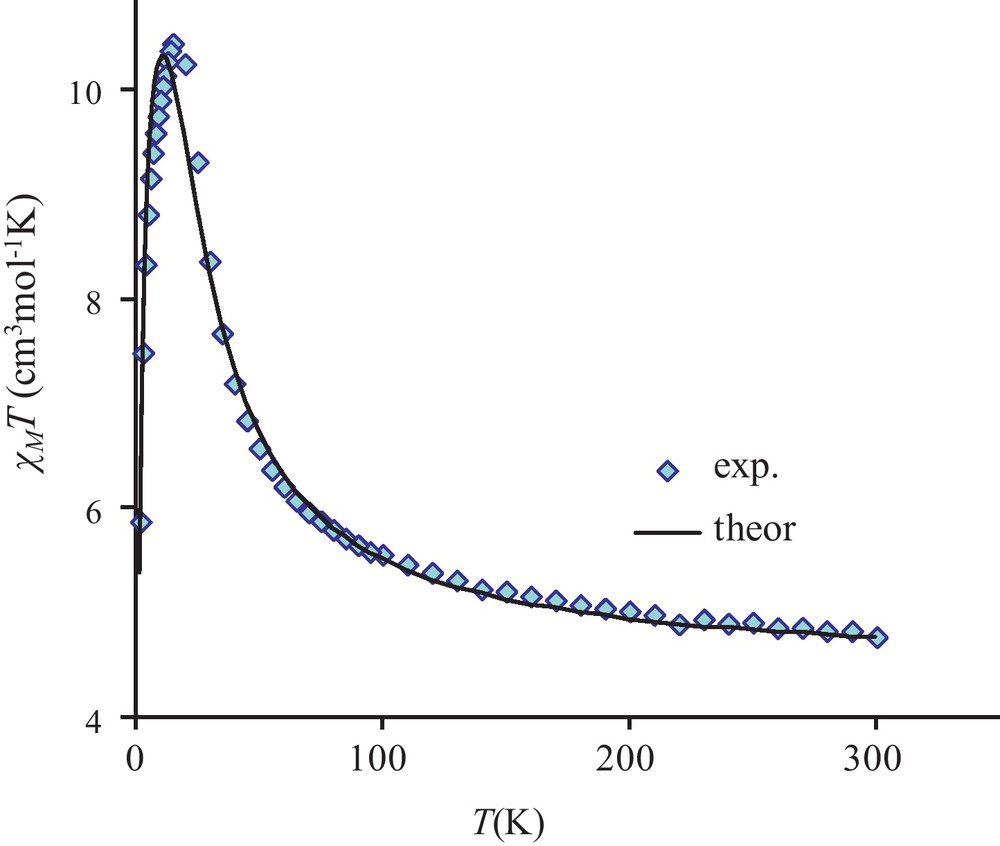
Temperature dependence of the χMT product for complex 3 under a 1 T applied field. Solid line corresponds to JNiNi = 3.9 cm−1, DNi = 7.6 cm−1, g = 2.11.
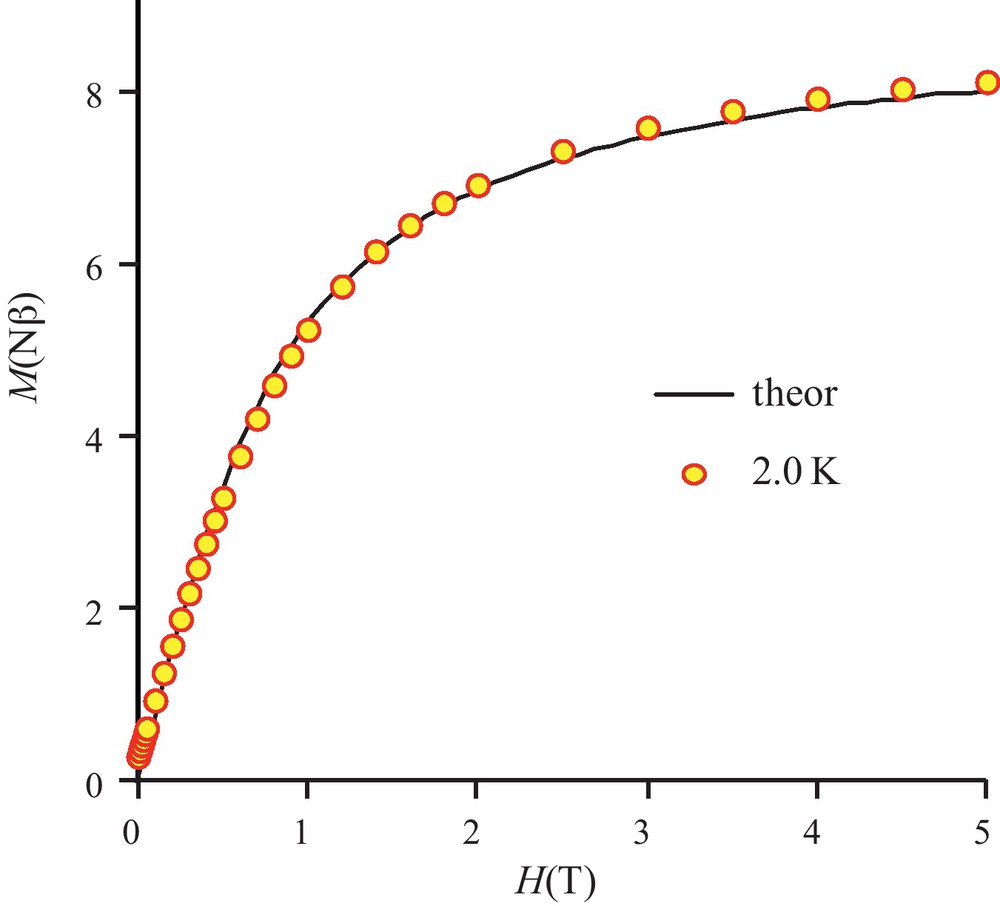
Field dependence of magnetization for complex 3. Solid line corresponds to JNiNi = 3.9 cm−1, g = 2.11, DNi = 4.0 cm−1, ENi = 0.3 cm−1.
To confirm the magnetic phase transition, alternating current susceptibility measurements have been investigated on complex 3. These ac susceptibility measurements show in-phase and out-of-phase signals, the latter being not frequency-dependent. Indeed the χ” maxima at 10, 200, 400 and 800 Hz (Fig. 7 and Fig. S2) centered around 13.5 K are characteristic of a three-dimensional weak ferromagnetic ordering. Unfortunately in-phase and out-of-phase signals are not observed when ac susceptibility measurements are made on the tetranuclear complex 1, which means that it cannot be considered as a single-molecule magnet.
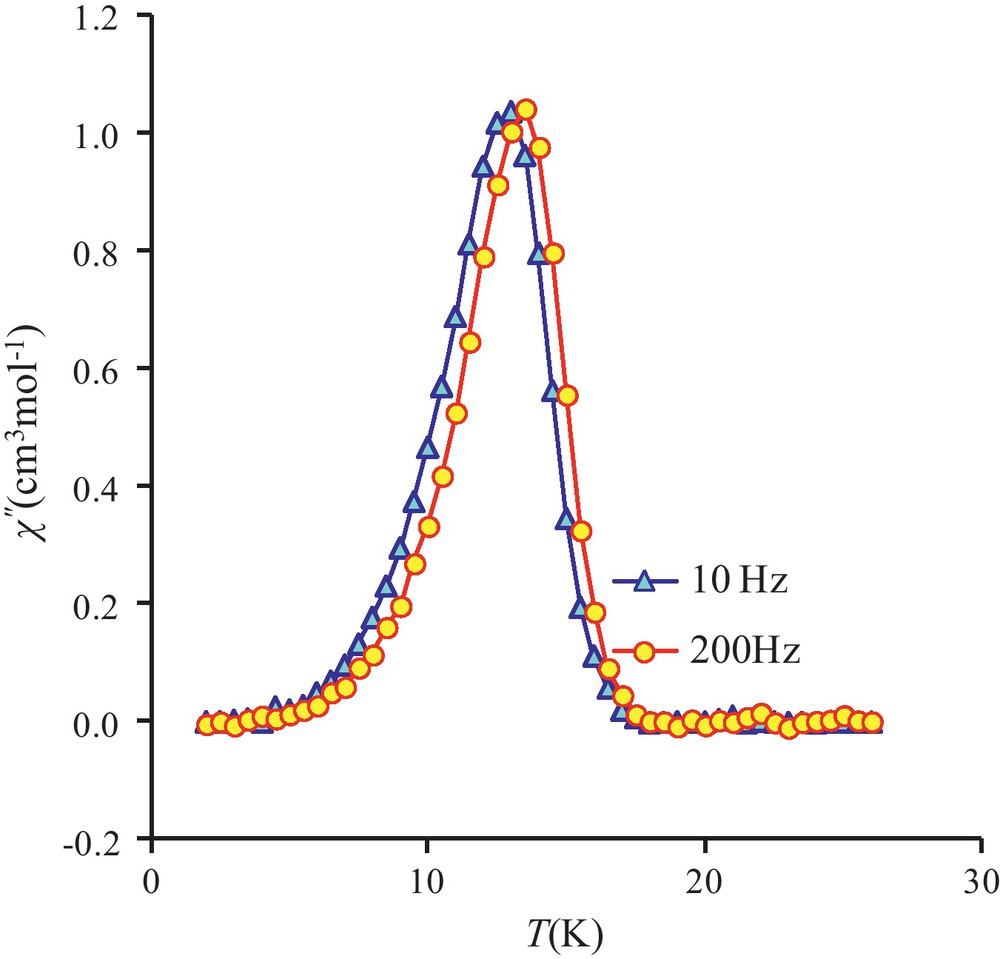
Out-of-phase susceptibilities (χ”) against temperature measured in a 2.7 G ac magnetic field oscillating at 10 (triangles) or 200 Hz (circles) frequencies for 3.
Similar observations have been made for complexes 4 and 5. Under a 0.1 T applied field, the χMT maxima observed at 14 K are respectively equal to 27.2 and 19.3 cm3 mol−1 K (Figs. S3 and S4) while these maxima decrease to 9.5 cm3 mol−1 K for measurements made with a 1.0 T applied field. Out-of-phase signals centered at 12 and 13.5 K are again observed (Figs. S5 and S6). On the contrary, complex 2 presents a slightly different behavior. Indeed the χMT difference at 1 and 0.1 T is much less important, 11.8 against 9.9 cm3 mol−1 K and the out-of-phase signal is weaker than in complexes 3, 4, 5, and centered at 8 K (Figs. S7 and S8).
3.3 Discussion
If the tetranuclear nickel cubane complex has been first prepared by serendipity, an experimental process allowing its preparation in a correct yield is reported. It belongs to the family of diketone or salicylaldehyde cubane complexes in which the four nickel ions are linked together by μ3-OMe bridges and with very similar Ni-O(OMe) bond lengths. As a consequence, the six Ni-Ni magnetic interactions can be considered as equivalent. Indeed, use of an Hamiltonian with two different J parameters does not give a better result. The structural determination also indicates that the Ni-O-Ni angles are quite homogeneous and vary from 96.41(5) to 97.87(5)°. The dependence of the interaction parameter JNiNi has been previously noted and bridging angle values lower than 100° are known to give ferromagnetic interactions, which explains the JNiNi value of 5.8 cm−1 found for complex 1 and the S = 4 ground state. In a recent work, a JNiNi value of 6.2 cm−1 has been deduced from an Hamiltonian that does not take into account the zero-field splitting parameter [18], what explains why our value (JNiNi = 5.8 cm−1) is slightly lower. On the contrary, the determination of the ZFS parameters is not so easy. While in mononuclear complexes the anisotropy can be usually enlarged by maximizing the geometrical distortions of the first coordination sphere and corroborated by ab initio calculations [30], in polynuclear complexes the anistropic intersite interactions are difficult to predict and hence the property of the whole system is far from being predictable. A recent reinvestigation of the axial zero-field splitting [31] allows to determine a tetragonality parameter Dstr by knowing the metal-ligand bond lengths and eventually an estimation of a Dmag term defining the Ni single-ion magnetic anisotropy. Applied to complex 1, the Dstr term of the Ni metal ion is equal to 10.6, that gives a Dmag value of 7.1 cm−1. This analysis confirms the presence of single-ion anisotropy in this complex, that cannot be determined in a straightforward way from experiment. On another side, the SHAPE program [32] indicates that the Ni coordination sphere is better described as a slightly deformed octahedron (SOh = 0.43), the S coefficient varying from zero for an ideal octahedron to 100. A slight octahedral deformation should be associated to low ZFS terms. Nevertheless, these two approaches of the ZFS problem do not disagree with the data calculated in the above section. Furthermore, the increase of the axial zero-field splitting term D on going from the tetranuclear cluster to the complex 3 strongly suggests that the three-dimensional arrangement must induce a larger deformation of the Ni coordination spheres.
The most original point developed in the present paper concerns the different ways used to assemble the tetranuclear Ni cubane units. Surprisingly, these Ni4 complexes are well separated from each other and there are no intermolecular hydrogen bonds in between these units. Furthermore these high-spin units with their ferromagnetic interactions and their S = 4 ground state do not behave as single-molecule magnets, what is confirmed by the absence of out-of-phase signals when alternating current (ac) susceptibility measurements are performed in the 2–25 K temperature range. A first way to associate such units consists in replacing the methanol molecules coordinated to the Ni ions by a diol molecule. Although we could not obtain crystals suitable for XRD study, analytical data of the resulting complex 2 do suggest that 1,3-propanediol links these Ni4 units for only two diol molecules are introduced in place of the four methanol molecules. This association is confirmed by ac susceptibility measurements performed in the 2–20 K temperature range. Observation of out-of-phase signals that are not frequency-dependent implies that magnetic ordering occurs. Unfortunately, these weak signals centered at 8 K appear on a large domain, from 6 to 10 K.
The situation becomes more interesting when amino-alcohol or amino-ethers are used, as in complexes 3, 4 and 5. The analytical data indicate that each methanol molecule is replaced by an amino-alcohol or amino-ether. The oxygen atom of the introduced ligands is coordinated to the nickel centre. This is demonstrated by the fact that primary amine RNH2 ligands are not able to replace methanol molecules of the Ni4 units and also by observation of visible absorption bands similar to those obtained with the starting complex 1. If the present cubane structure belongs to an old family of cubane units involving diketone or salicylaldehyde ligands, it presents the advantage of having at the periphery free methoxy groups that are prone to make hydrogen bonds with the introduced primary amine functions. Such a behavior has been observed in several metal complexes containing o-vanillin ligands [14,15]. Although we could not isolate crystals for XRD studies, the magnetic properties of complexes 3, 4 and 5, with the observed sharp χMT increase and the appearance of well-defined out-of-phase χ” susceptibility signals in the 12–14 K temperature range, evidence that self-assembling occurs. These signals cannot be associated to a SMM behavior for they are not frequency-dependent but they do confirm the presence of a three-dimensional magnetic ordering.
4 Conclusion
We have described in this work an experimental process that allows direct preparation of a tetranuclear nickel cubane derived from o-vanillin (2-hydroxy-3-methoxy-benzaldehyde) without hydrolysis of a Schiff base ligand. Contrary to what was previously published [16], this complex presents ferromagnetic Ni-Ni interactions with a S = 4 ground state. Magnetic susceptibility studies reveal that positive anisotropy terms DNi are active but ac susceptibility measurements confirm that the Dmol is not negative for the nickel cubane does not behave as a SMM. Replacement of methanol molecules coordinated to the Ni ions by a diol ligand such as 1,3-propanediol creates coordination bonds bridging these Ni4 entities through the diol ligand, as demonstrated by analytical results and ac susceptibility measurements.
Finally, it can be assumed that the interactions between tetranuclear units through a hydrogen-bonding network involving the pendant primary amine functions and the peripheral o-vanillin methoxy groups promote a three-dimensional ferromagnetic ordering when methanol molecules of the tetranuclear Ni4 complex 1 are replaced by amino-alcohol or amino-ether molecules.
Acknowledgements
This work was supported by the European Union sixth framework program NMP3-CT-2005-515767 entitled “MAGMANet: Molecular Approach to Nanomagnets and Multifunctional Materials”. G.N. thanks the Marie Curie Actions (FP7-People-IIF) for financial support. The authors are grateful to Dr A. Mari for technical assistance for the magnetic measurements.
Appendix A Supplementary data
Temperature dependence of the χMT product for complex 3 at different applied magnetic fields: 0.1 T (circles), 0.7 T (triangles), 1.0 T (squares), 2.0 T (diamonds) and 5.0 T (stars). In-phase (χ’) and out-of-phase susceptibilities (χ”) against temperature measured in a 2.7 G ac magnetic field oscillating at 200 (squares), 400 (triangles) and 800 Hz (circles) frequencies for 3. Temperature dependence of the χMT product for complex 4 at 0.1 T. Temperature dependence of the χMT product for complex 5 at different applied magnetic fields: 0.1 T (×) and 1 T (triangles). Out-of-phase susceptibilities (χ”) against temperature measured in a 2.7 G ac magnetic field oscillating at 10 (triangles) and 200 Hz (squares) frequencies for 4. Out-of-phase susceptibilities (χ”) against temperature measured in a 2.7 G ac magnetic field oscillating at 10 (triangles) and 200 Hz (squares) frequencies for 5. Temperature dependence of the χMT product for complex 2 at different applied magnetic fields: 0.1 T (×) and 1 T (triangles). Out-of-phase susceptibilities (χ”) against temperature measured in a 2.7 G ac magnetic field oscillating at 10 (triangles) 200 (circles) and 1000 Hz (squares) frequencies for 2.