1 Introduction
Graphite intercalation compounds (GICs) are original low-dimensionality materials that allow extensive studies in order to associate chemical and structural properties with the variation of the physical properties. For example, the discovery of superconductivity in CaC6 and YbC6 graphite intercalation compounds (GICs) with high critical temperatures for this class of lamellar compounds (TC = 11.5 K and 6 K, respectively) [1] leads to numerous studies in order to understand as far as possible these compounds. For such a work, it is obvious that the quality of the sample is, of course, a key point for physical studies. Chemical and structural defects must be, as far as possible, avoided in the final GIC. So, the prepared samples have to be intercalated in the bulk, with an as important coherence length along the c-axis and in the ab plane as possible. With this aim, we have developed a synthesis route allowing the preparation of bulk compounds. The first works involved the use of liquid alloys containing sodium or heavy alkali-metals, leading to the formation of original ternary bulk GICs [2,3]. Then, due to the huge interest of the scientific community regarding intercalation of lithium into graphite, we naturally oriented our studies using lithium as intercalation vector [4]. This procedure is based on the reaction between a pyrolytic graphite platelet hung on a tungsten sample-holder and a liquid alloy containing lithium and another metallic element. We evidenced the role of lithium as an efficient intercalation agent for various elements such as alkaline-earth or lanthanide metals that hardly intercalate alone into graphite [5,6]. On the one hand, it allows one to considerably reduce the reaction temperature in order to avoid the parasitic formation of carbide and the destruction of the lamellar lattice of graphite. On the other hand, it has been evidenced that lithium atoms intercalate first, leading to a pre-opening of the graphitic galleries. Then it makes easier the intercalation of the second metal with the formation of binary or ternary GICs.
The preparation of binary compounds with the complete evacuation of lithium atoms from the Van der Waals gap is an originality of the solid-liquid reaction in lithium liquid alloys. Indeed, in the case of reactions performed in potassium-based alloys, only the formation of graphite-potassium or graphite-potassium-third element GICs has been observed. In these systems, potassium does not only play the role of an intercalation vector but is always at least co-intercalated into graphite. Contrarily, in some systems lithium clearly acts only as a vector, allowing the preparation of original binary bulk compounds of the alloyed element, very interesting for structural and physical properties.
Considering the specificity of lithium, many attempts at intercalation of electronegative elements or fairly electronegative elements have been made [7]. More recently, we tried to intercalate various metals (alkaline-earth metals Mg, Ca, Ba and Sr; rare earth metals Sm, Eu and Yb) that were only superficially intercalated into graphite by action of the vapor phase [5,6].
Regarding the thermodynamic Li-M binary phase diagrams, the composition of the alloy and the reaction temperature have been chosen to perform reactions in a homogeneous liquid metallic alloy. Then, various reaction times have also been tested for intercalation reactions. Various actions of the alloy towards graphite have been noticed.
Whatever the third element considered, if the alloy is not rich enough in lithium, no intercalation occurs. Contrarily, if the alloy is mainly composed of lithium (> 80 at.%) only LiC6 can be formed. Finally, for intermediate compositions (between 50 and 80 atomic percent in lithium), the synthesized compound depends on the system considered.
When the third element is Mg, Sr, Sm or Yb, only lithium can be intercalated into graphite, leading to first-stage or second-stage lithium-GICs depending on the experimental parameters. No other binary or ternary graphite intercalation compound has been observed in these systems, even if the existence of SrC6, SmC6 and YbC6 GICs are mentioned in the literature [5,6].
In the graphite-lithium-barium system, it has been possible to prepare LiC6 or BaC6 GICs, but no ternary compound has been pointed out [8].
Finally, the experiments performed in the graphite-lithium-calcium (G-Li-Ca) and graphite-lithium-europium (G-Li-Eu) systems have highlighted an easy intercalation of the element alloyed to lithium. Binary and ternary GICs have been evidenced in these systems. This equivalent behavior regarding the intercalation reactivity toward graphite must be considered together with the large similarities of the atomic properties of metallic calcium and metallic europium. Each metal shows a possible but limited ability to be intercalated alone in the vapor phase. They both expose a low electronegativity value considering the Pauling scale (χCa = 1.0 and χEu = 1.1) and their atomic radii are very close (rCa = 197.4 pm and rEu = 199.5 pm). Last, they are both able to stabilize their corresponding divalent ion.
Based on previous studies, the present review aspires to emphasize the differences and analogies between MC6 binary compounds obtained in these systems. Moreover, the recent studies performed on the various synthesized ternary compounds of these systems are also presented in order to highlight common points (for example, their poly-layered stacking sequences) and differences regarding their physical properties.
2 Material and methods
Reagents must be very pure to synthesize graphite intercalation compounds. Indeed for solid-liquid reactions, if other constituents are present (i.e. impurities) the thermodynamic conditions in a given system are strongly modified. The distilled calcium of dendritic nature exhibits a purity of 99.99%. The commercial grade lithium (purity 99.95%) is further purified by heating, in a glove box under argon atmosphere, at around 200 °C, a temperature higher than the melting point of pure lithium. In this way, the impurities float on its surface and are eliminated by a mechanical sweep. Commercial grade of metallic europium is of purity higher than 99.9%.
X-ray diffraction experiments are performed using a θ/2θ diffractometer with a molybdenum anticathode (λMoKα1 = 70.926 pm) by recording the 00l reflections, or using the rotating crystal method.
The elementary compositions of the different GICs have been established by nuclear microprobe analysis, allowing the simultaneous quantification of light and heavy elements in the studied systems [9]. Magnetic susceptibility and magnetization measurements on europium-based GICs have been performed with a Physical Properties Measurement System, Quantum Design (PPMS) whereas the measurements concerning superconducting compounds have been investigated thanks to a Superconducting QUantum Interference Device (SQUID) apparatus. Physical properties of the bulk compounds have also been probed using μSR spectroscopy. Further details concerning the last method can be retrieved in [10].
3 Synthesis, intercalation mechanism and chemical compositions
In the graphite-lithium-(calcium or europium) systems, binary and ternary compounds were obtained from HOPG pristine graphite platelets as host. The synthesis is carried out by immersing graphite samples hung on a tungsten sample-holder in a liquid lithium-based alloy of a well-chosen composition at a temperature allowing the melting of the alloy. More details about the synthesis are given in [4].
Contrarily to reactions performed with lithium-calcium alloys, those carried out in lithium-europium alloys needed preliminary experiments to determine the melting temperature of the alloy since the corresponding phase diagram remains unknown. With this aim, we performed DSC experiments by conditioning various lithium-europium alloys in stainless steel crucible sealed under argon. Such experiments revealed that even if lithium is melted and dissolves some metallic europium, we can expect the presence of residual europium solid particles as the liquidus equilibrium line of the Eu-Li binary phase diagram is not reached.
In order to follow the intercalation mechanism in both systems, numerous reactions were carried out and each reaction product was investigated by ex situ XRD experiments. The temperature and composition conditions of the alloys involved are those leading to the synthesis of the binary and ternary compounds in the graphite-lithium-(calcium or europium) systems. Products obtained with reaction times from 20 min to 10 days – when the thermodynamic equilibrium is reached – have been systematically studied [11,12].
For all compounds, lithium intercalates first, pre-opening the Van der Waals gaps of pristine graphite thus allowing the intercalation of the alloyed metal. However, the mechanism leading to the MC6 binary compounds (M = Ca or Eu) depends on the system considered.
For CaC6, a mixture of high-stage Li-GICs is observed. In a second step, LiC6 (1st stage GIC) is obtained, and consecutively calcium progressively replaces lithium until the formation of pure CaC6. This constitutes a well-defined multi-step intercalation mechanism.
For EuC6, the first step is the intercalation of lithium atoms, but graphite is not saturated and a stage-2 Li-GIC is formed. Consecutively, Eu atoms are intercalated into the graphitic host simultaneously with lithium ones, leading to a LiC6 - EuC6 mixture. During the last step, lithium is substituted by europium and the binary EuC6 compound constitutes the stable final phase. However, the residual presence of lithium inclusions in the bulk EuC6 sample has been pointed out. Contrarily, no similar inclusions have been observed in the CaC6 compound. The intercalation mechanism noted in the G-Li-Eu system appears different from that of the G-Li-Ca system.
The possible formation of ternary compounds including lithium for these systems consists in an important similarity. For such cases, intercalation mechanisms are quite the same: preliminary intercalation of lithium occurs, and consecutively the third element is intercalated in the same Van der Waals gaps as lithium. It should be noted that such ternary GICs have never been observed in other G-Li-M systems.
The different possible formation mechanisms are summarized on Fig. 1.

Schematic representation of the various formation mechanisms involved in the synthesis of binary and ternary calcium- or europium-based GICs. First step corresponds to the intercalation of lithium forming high-stage compounds. Then, three possibilities are observed: c1: LiC6 is formed then (d1) calcium atoms substitute lithium ones, leading to CaC6; c2: lithium and europium atoms are intercalated simultaneously; d2: then lithium is evacuated leading to bulk EuC6; c3: LiC6 is formed then (d3) calcium or europium is added, leading to ternary compounds.
All chemical compositions of the binary and ternary compounds were determined using ion beam analysis thanks to the nuclear microprobe in Saclay. This technique presents a huge advantage as it allows the simultaneous quantification of lithium by nuclear reaction together with heavier elements (C, Ca, Eu) by Rutterford Back Scattering [9]. Binary compounds were studied to ensure that the total evacuation of lithium atoms from the samples occurred, what is observed for graphite-calcium binary compound which is characterized by less than 1 atomic percent of residual lithium. Moreover, the analysis confirms, of course, the CaC6 formula. The result is surprisingly different for the graphite-europium compound even if it has been demonstrated that EuC6 compound is clearly obtained by the solid-liquid method [13]. Nuclear microprobe experiments show a significant content of lithium with a composition close to Li0.4-EuC6 where lithium is present as inclusions between EuC6 zones. This assumption is confirmed as no lithium-graphite intercalation compound has been detected by X-ray diffraction.
Concerning ternary compounds, ion beam analyses were carried out to determine the exact formulas of the GICs. Hence, ternary compounds in the G-Li-Ca system present the formulas Li3Ca2C6 and Li0.5Ca3C6, whereas the ternary GIC of the G-Li-Eu system exposes a composition close to Li0.25Eu1.95C6. Finally, it should be noted that all the ternary GICs in both systems exhibit a homogeneous chemical composition laterally, as well as in depth, without any elementary segregation, which differs from the case of binary compounds.
4 Crystal structure
Crystal structures of both MC6 compounds have been resolved. Even if the stoichiometry and planar symmetries of these GICs are the same, these compounds do not crystallize in the same space group due to a different occupation of the prismatic sites generated by the strict superimposition of two successive graphene layers, leading to different c-axis stacking sequences for the intercalated sheets (Fig. 2). For CaC6, the three sites are successively occupied, with AαAβAγAαAβAγ… stacking. Then the c parameter corresponds to three times the repeat distance: c = 3 Ic (with Ic = 452 pm) corresponding to a rhombohedral symmetry with a R-3 m space group [11]. In the case of EuC6, only two sites are alternately occupied, the stacking is then AαAβAαAβ… and c = 2 Ic (with Ic = 487 pm) leading to a hexagonal crystal symmetry with a P63/mmc space group [13].
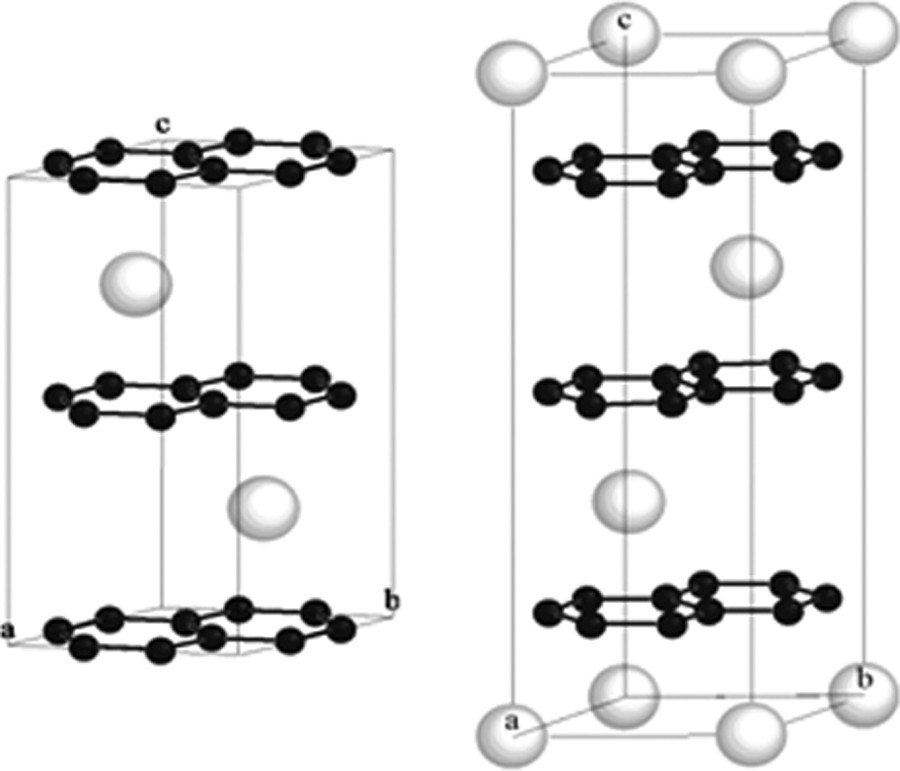
Unit cells of EuC6 crystallizing in the P63/mmc space group (left) and CaC6 (R-3 m) represented in hexagonal configuration (right).
The determination of the structural properties of ternary compounds remains one of the challenges in the field of the crystal chemistry of graphite intercalation compounds. In many cases, they remain unsolved. However, the collected crystallographic data allow emphasizing some similarities between both systems, as they all exhibit a poly-layered intercalated sheet. Moreover, as lithium is the most electropositive intercalated metal, Li planes are always adjacent to graphene sheets (richer in electrons than pristine graphene) and surround calcium or europium planes. The difference is the nature of the layer(s) in the middle of the intercalated sheet. For calcium-based compounds, one or three lithium planes settle the intercalated sheet. The repeat distances range then from 776 pm (Li3Ca2C6) to 970 pm (Li0.5Ca3C6) [14]. In the ternary compound belonging to G-Li-Eu system, europium is present at the center of the intercalated sheets, with a repeat distance close to 800 pm [15]. Unfortunately, the three-dimensional crystal structures have not yet been determined.
5 Physical properties
Depending on the element alloyed with lithium, GICs’ magnetic properties will be considerably different. Indeed, the possible existence of divalent europium characterized by a high magnetic moment (μeff = 7.94 μB) is able to confer remarkable magnetic properties to europium-based compounds, which is obviously not the case for calcium. However, for a given system, analogies appear clearly between binary and ternary compounds.
Regarding to the G-Li-Ca system, Li0.5Ca3C6 is a non-magnetic material. Contrarily, CaC6 binary compound and the Li3Ca2C6 ternary one exhibit superconducting properties below critical temperatures of 11.5 K and 11.15 K, respectively [12]. Both compounds appear as type II superconducting materials with a weak anisotropy of the critical fields [16,17]. The binary compound has been intensively studied by various techniques such as magnetisation measurements, low/high frequence electrodynamic measurements [18,19], scanning tunnelling spectroscopy [20–22], resistivity and magnetisation measurements versus temperature/pressure [23–26], determination of the specific heat [27], angle-resolved photoemission spectroscopy [28], muon spin spectroscopy [29,30]. These experiments agree with an “s-wave” symmetry of the gap function, compatible with the standard Bardeen-Cooper-Schrieffer (BCS) theory.
In the G-Li-Eu system, binary and ternary compounds are characterized by remarkable magnetic properties. Indeed, EuC6 extensively studied by numerous techniques [31–35], exhibits a well-known antiferromagnetic transition with a Néel temperature of 40 K. Contrarily, the magnetic behaviour of the ternary compound appears rather more complex and has been studied using usual magnetic measurements, but also using muon-spin and 151Eu Mössbauer spectroscopies. The assessment of the different magnetic interactions in the structure due to different europium sites is partially derived from those identified for the binary compound. The main transition, occurring at 225 K, is ferromagnetic and is due to corresponding interactions along the c-axis between main europium planes belonging to the same intercalated sheet [5]. Below 200 K, antiferromagnetic interactions are then detected, clearly pointed out under 125 K. Finally, another transition occurs around 45 K. A possible frustration phenomenon detected under 150 K is also pointed out. Some physical properties relative to binary and ternary compounds determined in this study are schematically compared on Fig. 3.
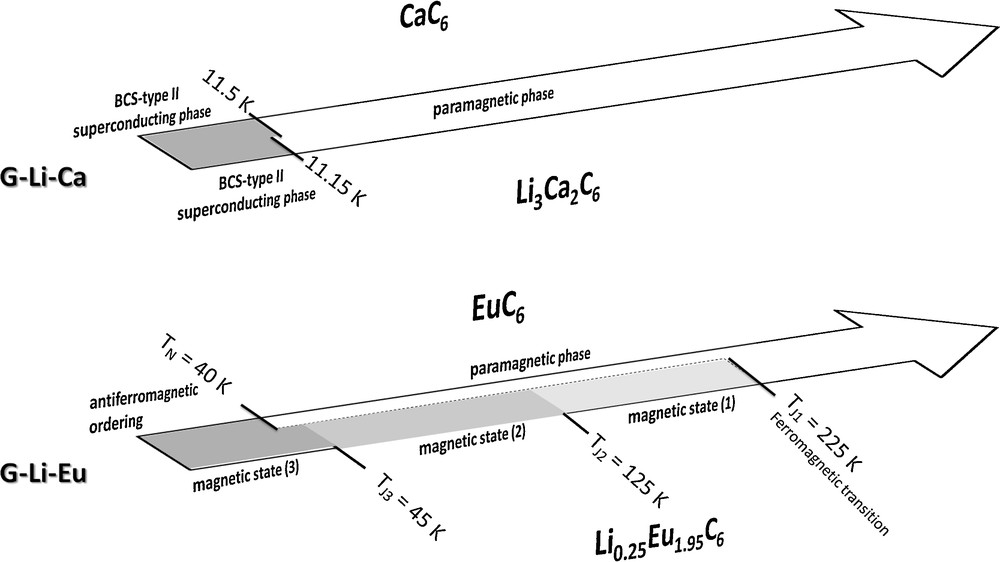
Comparative evolution versus temperature of the magnetic properties determined for binary and ternary compounds obtained in the G-Li-Ca and G-Li-Eu systems.
6 Conclusions
We have shown that binary and ternary compounds are synthesized in both cases with similar reaction conditions, but many properties of these compounds are amazingly different. For CaC6 and EuC6 binaries, the 2D unit cells are the same, but their c-axis stacking sequences lead to different space groups corresponding to rhombohedral and hexagonal symmetries, respectively. Even if the formation mechanism of such compounds appears quite similar, some differences are pointed out. Otherwise, their physical properties are obviously strongly different due to the nature of the intercalated species. Concerning ternary compounds belonging to both systems, the main common point is their c-axis poly-layered stacking sequences but some huge differences are revealed in their physical properties.
Acknowledgments
The authors thank Christine Bellouard, Gianrico Lamura, Pascal Berger and Ghouti Medjhadi for fruitful discussions and implication in the various measurements.