1 Introduction
Toxic heavy metal discharge is infecting the atmosphere day by day. Finding out ways to minimize their harmful effect is a challenging task for scientists. Copper is a heavy metal widely present in nature. It is a soft transition metal and constituent of alloys, pharmaceutical products and found in almost all body tissues [1]. The suggested safe limit by WHO in drinking water for copper is 2 ppm [2]. Its excess has a deleterious effect on living organisms. Copper toxicity is based on its oxidation state: Cu(I) and Cu(II) [3]. It can pollute the environment through various means. Different industries, such as mining, paper, refining, petrochemical, metallurgy and fertilizer dispose copper in their effluents [4]. Therefore, the workers of these industries are more exposed to copper toxicity [5]. Corrosion of water supply pipes also releases it into aqueous streams [3]. Prolonged copper exposure is associated with a number of health problems, including jaundice, liver dysfunction, headache, nausea, diarrhea, stomach and intestinal problems, depression, learning disorders, fatigue, kidney and brain damage [6,7]. Wilson's disease is a hereditary disorder in which the body is unable to metabolize copper. Thus, patients suffering from this disease accumulate copper both by food and environmental sources. Hence, they are affected badly by copper toxicity [7]. Marine life also reveals severe effect of copper contamination. It distorts the normal functioning of gills, liver, kidneys, smell organs and the nervous system in them [8]. Furthermore, in waste water, it hinders the biodegradation of organic pollutants and hence, aid in increasing pollution. Therefore, it is of prime importance to search for methods which effectively remove copper from industrial discharge prior to its dumping [9].
Among the numerous techniques applied to eliminate copper from the atmosphere are biosorption [10], adsorption [11], ion exchange [12], electrochemical treatment [13], chemical precipitation [14] and solvent extraction [15]. In this connection, liquid membrane (LM) is emerged as a truly green technology. The most prominent features of LM are cost effectiveness, less laboriousness, easy handling, specificity, and use of fewer amounts of expensive carrier as well as organic liquids. It has low carrier to metal solution ratio [16–19].
Supported liquid membrane (SLM) is a type of LM mostly used in industries. An organic liquid with carrier supported on the membrane is sandwiched between the two aqueous phases. Carrier solution is impregnated on support membrane due to capillary forces. Despite the proper choice of solvent and membrane, carrier leaching during the passage of time is still a challenging difficulty [20–23]. Therefore, new technological advancements are carried out in SLM to overcome membrane instability and for its wider application in separation fields. Typical applications of SLM are recovery and separation of precious metals [24], radioactive metals [25], rare earth metals [26], toxic metals [20,22], heavy metals [6], sugar [27], dyes [28], and drugs [29].
Carrier plays a key role in specific membrane separation. Recently, macrocyclic carriers have been explored and found efficient as well as selective towards ionic and neutral guests. Calixarenes are popular vase-like macromolecules capable of forming inclusion complexes with ions and neutral species. Their derivatives can be easily synthesized by adding the desired functionality [30–34]. In LM transport, many calixarene derivatives have been employed successfully [16–21,35–38]. Herein, we report the SLM study of p-morpholinomethylcalix[4]arene (1), as shown in Fig. 1 for Cu(II), whose selectivity has been previously evaluated by our group [33]. Celgard 2400 and 2500 membranes have been used as the solid support in SLM. The effect of the fundamental parameters influencing the transport process, e.g. carrier concentration, type of solvent used in the membrane, pH of donor and acceptor phases, anion types and dipping time for membrane preparation has been investigated. Danesi mass transfer model was used to calculate the permeability coefficients for each parameter studied. The diffusion coefficient was obtained experimentally using the Reinhoudt's model, lag time method and by Wilke–Chang relation and compared.
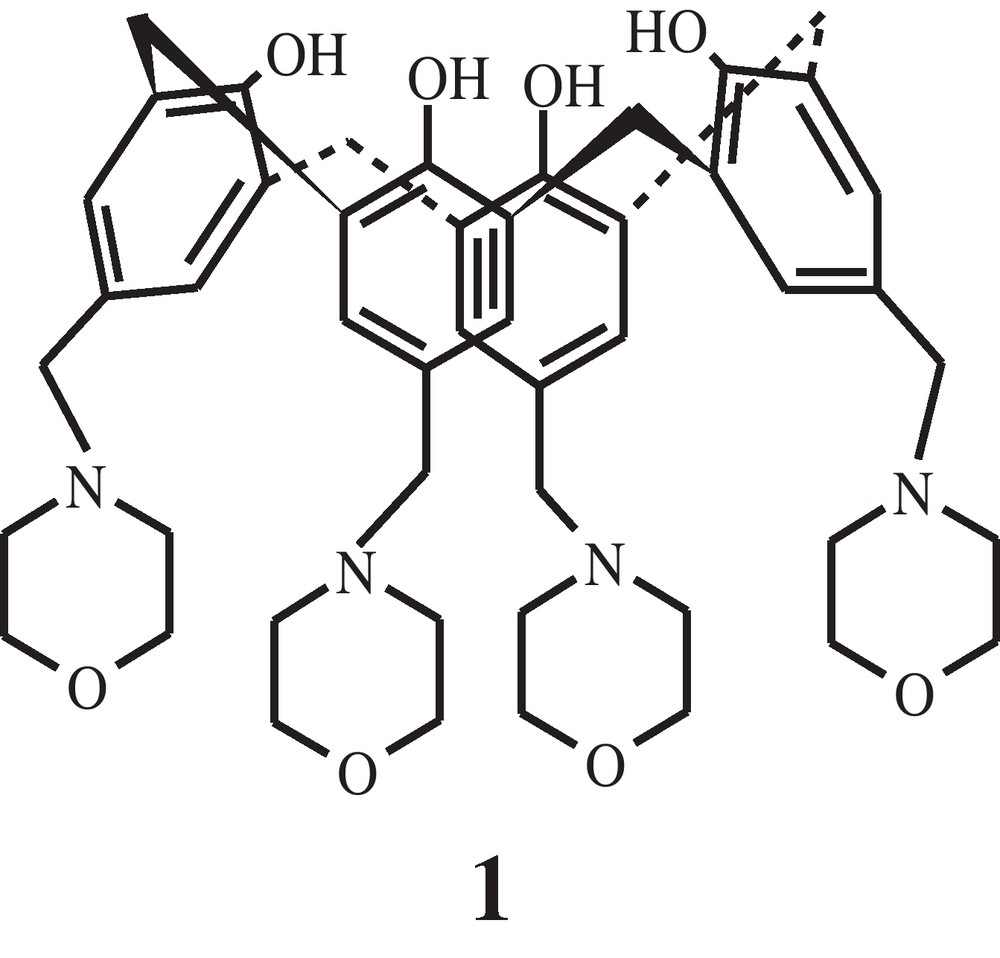
p-Morpholinomethylcalix[4]arene (1).
2 Experimental methods
2.1 Materials
Analytical grade reagent chemicals were used to make aqueous solutions. Ultra pure deionized water for the solution preparation was taken from a Milli-Q system (ELGA Model CLASSIC UVF, UK). The 1 was synthesized following the method reported in [33]. The solid polymeric membranes, Celgard 2500 (thickness: 25 μm, porosity: 45%) and Celgard 2400 (thickness: 25 μm, porosity: 38%) were provided by Selcuk University, Department of Chemistry, Konya, Turkey and AMTech, Islamabad, Pakistan.
2.2 Apparatus
The cell used in the present study for SLM was made from Perspex material as presented in Fig. 2. It consisted of two cylindrical portions of equal size with volume capacity of 106 mL each. The support membrane was clamped between the two portions with flanges. Both portions were equipped with electrical stirrers at a speed greater than 1000 rpm. The inlet and outlet openings were given to fill and drain donor and acceptor solutions, respectively. The effective membrane exposing area was 12.56 cm2. The membrane impregnated with 1 is used here. Cu(NO3)2 solution and deionized water were used as donor phase and acceptor phase, respectively. Temperature was kept constant for all experiments, i.e. 25 °C. Conductivity change was analyzed periodically with the help of a conductivity electrode placed in the acceptor phase. The experiments were repeated twice for each parameter and the experimental error was calculated.

Supported liquid membrane apparatus for Cu(II) transport.
2.3 Analytical instruments
The conductivity of the solutions was checked with Inolab Cond 720 conductivity meter. Scanning electron microscope (SEM) images were obtained by Model JSM-6490 A (Jeol Japan). The solutions pH was maintained with 781-pH/ion meter (Metrohm Switzerland). Stirring was accomplished by using a mechanical shaker (Gallenkamp) with temperature controller. Perkin Elmer Lambda 35 UV/Vis spectrophotometer was used to measure the solution absorbance.
2.4 Membrane preparation
The solution of 1 (1 × 10−3 M), in diphenyl ether, was prepared to soak Celgard membrane for 60 min. Membrane pores were filled through capillary action by this solution. The impregnation of 1 onto the membrane results in an increase in its weight, which confirms the loading of 1. Soft tissue paper was used to absorb extra solution from the membrane surface and kept in open atmosphere for few minutes so that it could dry well. Afterwards, the membrane was clamped in the SLM apparatus.
2.5 Solvent extraction
Cu(NO3)2 solutions (10 mL each) of concentration 2.5 × 10−5 M were used and their pH was maintained in the range of pH = 1–6, using HCl. Cu(NO3)2 was extracted with chloroform solution of 1 (1 × 10−3 M, 10 mL) at 298 K in a stoppered glass flask with a mechanical shaker for 60 min. The changes in the absorption spectra of Cu(NO3)2 solutions after extraction were checked by a spectrophotometer.
2.6 Solvent decomplexation
The extracted chloroform solutions (10 mL) from each acidic Cu(NO3)2 solutions were briskly stirred in a stoppered glass flask with a mechanical shaker for 60 min with 10 mL of HCl (pH = 5–6)/NaOH (pH = 8–10) at 298 K. After this extraction, the changes in absorption spectra of HCl and/or NaOH solutions were tested by a spectrophotometer.
3 Supported liquid membrane transport
A membrane is frequently evaluated by the permeability (P) and flux (J) values.
3.1 Permeability (P)
Permeability is defined as a quantity of metal transferred through a specific surface area of membrane per unit time. Danesi [20,21] mass-transfer model is followed to calculate permeability (P) values from these equations.
(1) |
(2) |
3.2 Flux (J)
The flux is the rate of metal transport through the membrane. The transport of Cu(II) complexed with 1 is supposed to be diffusive in nature, therefore, flux (J) values were calculated using the following equation,
(3) |
4 Results and discussion
Cu(II) transport efficiency across SLM using 1 from its salt solution has been examined. The effect of different parameters, such as solvent, membrane dipping time, support membrane, co-anions, pH of aqueous phases and carrier concentration on Cu(II) permeation were optimized to achieve better separation.
4.1 Scanning electron microscopy characterization
The comparison of SEM images of Celgard 2500 membrane before and after carrier impregnation was given in Fig. 3a and b, respectively. Membrane morphology clearly showed that the membranes pores become narrower when immersed in the carrier solution.

Scanning electron microscopy images of Celgard 2500 membrane (a) before impregnation and (b) after impregnation.
4.2 Effect of solvent on Cu(II) transport
Membrane solvent plays a key role in the SLM permeation process. The characteristics features of solvents used in SLM are polarity, viscosity, water miscibility, volatility and surface tension. The solvent polarity favors salt partition and thus, helps in extraction into organic phase. More viscous liquids form stable membrane but it hinders high mass transfer; therefore, less viscous solvents are preferably used. Solvent immiscibility in water is also a vital property because the ratio of membrane and aqueous solutions is quite low. Volatility also affects membrane stability; less volatile liquids make stable membrane. As solvent resides in the membrane pores due to capillary forces, therefore, surface tension should be high enough for it to stay for a long time in the membrane. Consequently, in order to achieve efficient transport efficiency, solvent should be more polar, less viscous, water-immiscible, less volatile and with high surface tension. At a glance, it can be understood that a compromise in solvent property is made to balance membrane permeation and stability [39].
In the present study, the effect of solvent on Cu(II) transport across SLM was checked by keeping as donor phase the Cu(NO3)2 (1 × 10−2 M) solution, deionized water as acceptor phase, 1 (1 × 10−3 M) solution, Celgard 2500 membrane and maintaining the temperature at 298 K. On the basis of compound 1 solubility data that were collected previously in BLM study [19], chloroform and diphenyl ether were selected as membrane solvent in this work because 1 was found insoluble in carbon tetrachloride, butanol, ethyl acetate, 1,2-dichloroethane, xylene, n-hexane and diethyl ether. The permeability (P) and flux (J) values as well as the physicochemical properties of solvents are listed in Table 1. From the results, it is observed that P and J values of diphenyl ether are higher than those of chloroform. The comparison of viscosity values reveals that diphenyl ether is more viscous than chloroform and hence, makes stable membrane. Despite of having electronegative oxygen, dielectric constant value of diphenyl ether is less than that of chloroform indicating that polarity of C–Cl bond is more than that of C–O. Due to the high polarity, chloroform cannot make stable membrane. Surface tension value of diphenyl ether is relatively high as compared to other solvents. Lastly, vapor pressure and boiling point values show that diphenyl ether is less volatile, which is yet another quality for making stable membrane. Diphenyl ether is not only water immiscible; but it is also a significantly polar solvent to solvate ions as well as to make a stable membrane. Therefore, it exhibits high P and J values as compared to chloroform. This observation is also favored by the fact that mostly nitrophenyl octyl ether and nitrophenyl hexyl ether are employed in SLM study and give distinguishing transport [16,20,21,35–38].
Kinetic parameters of Cu(II) in different solvents with their physicochemical characteristics (Carrier = 1 × 10−3 M, Cu(NO3)2 = 1 × 10−2 M as donor phase, Celgard 2500 membrane, 298 K) [47].
Solvent | P × 106 (m/s) | J × 105 (mol/m2/s) | η (cP) | ɛ0 | γ (N/m) | Vapour pressure (Pa) | Boiling point (°C) |
Chloroform | 3.77 ± 0.5 | 0.881 ± 0.01 | 0.58 | 4.81 | 0.027 | 30000 | 61.2 |
Diphenyl ether | 6.22 ± 0.7 | 1.86 ± 0.08 | 3.34a | 3.90 | 0.038 | 133.3 | 257 |
a [48].
Many researchers are applying vegetable oil as solvent in SLM recently because they are non-toxic, environmentally friendly, biodegradable and economical as compared to conventional organic solvents used in SLM. Herein, the solubility of 1 was checked in coconut oil for this purpose but it was found to be insoluble. The variation in concentration of Cu(II) in both aqueous phases against time is plotted in Fig. 4. Initially, Cu(II) concentration decreases sharply in donor phase accompanied by an increase in acceptor phase. After some time, both curves become linear, indicating that Cu(II) permeation is about to end.
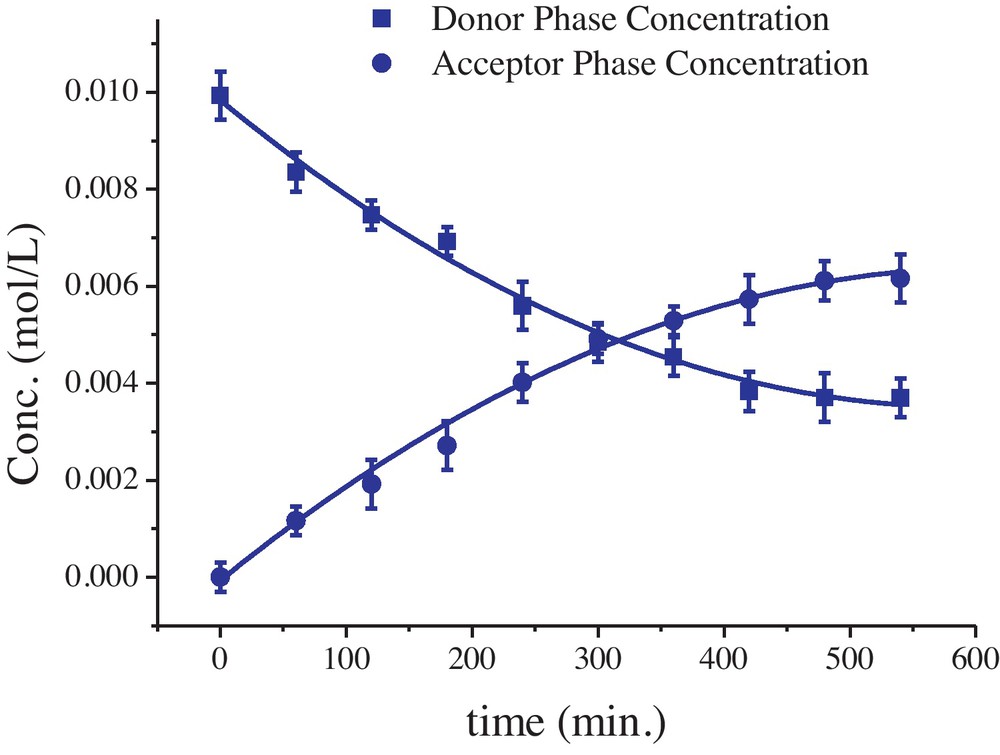
Variation in Cu(II) concentration through supported liquid membrane transport with time. Donor phase: Cu(NO3)2 = 1 × 10−2 M, membrane phase: 1 × 10−3 M carrier in diphenyl ether with Celgard 2500 membrane, acceptor phase: deionized water at 298 K.
4.3 Effect of membrane dipping time
Investigating membrane dipping time was aimed to check the time for membrane preparation. Membrane preparation procedures also play a significant role in transport phenomena. Therefore, membrane dipping time was varied from 1 to 27 h to observe its effect on Cu(II) transport. Through the study all the other conditions, i.e. donor phase of 1 × 10−2 M Cu(NO3)2 solution, deionized water as acceptor phase, 1 × 10−3 M 1 in diphenyl ether, Celgard 2500 membrane and at 298 K were kept the same and the results were presented in Table 2. Generally, membrane is dipped in the carrier solution for 24 h but as it is clear from the results that 1 h dipping is sufficient here and because permeability (P) and flux (J) values are approximately the same whether we dip membrane for 1 h or 27 h, therefore 1 h is optimized for further study. The possible explanation for this fact can be attributed to the viscosity and solvating property of diphenyl ether compared to chloroform. It has also been noticed that diphenyl ether fully saturates the membrane with the carrier within 1 h.
Kinetic parameters of Cu(II) at different membrane dipping time (Carrier = 1 × 10−3 M in diphenyl ether, Cu(NO3)2 = 1 × 10−2 M as donor phase, Celgard 2500, 298 K).
Membrane dipping time (h) | P × 106 (m/s) | J × 105 (mol/m2/s) |
1 | 6.22 ± 0.7 | 1.86 ± 0.08 |
17 | 6.41 ± 0.5 | 1.44 ± 0.06 |
27 | 6.97 ± 0.6 | 1.71 ± 0.09 |
4.4 Effect of support membrane on Cu(II) transport
The impact of support membrane on SLM process is fundamental because membrane stability along with other factors relies on it. Membrane hydrophobicity, porosity, tortuosity, mechanical strength, thickness, chemical reactivity and cost are some declaring properties essential to acquire suitable support membrane. The hydrophobic nature gives membrane stability in aqueous solution for long time use and also makes it compatible for organic solution in its pores. Porosity and tortuosity of membrane has an influential effect on membrane flux and permeability because organic solvents are held within the membrane pores. Therefore, interfacial tension between aqueous and membrane phase, which always tries to expel organic liquid from membrane pores, is minimum in small pores [39]. Consequently, a highly porous membrane with small pore size is a better choice for noticeable metal transport in SLM. Hence, in the present investigation, Celgard 2500 and Celgard 2400 polymeric polypropylene membrane were selected as support to check their affect on Cu(II) transport. For this study, the initial concentration of Cu(NO3)2 in donor phase was 1 × 10−2 M and 1 concentration in diphenyl ether at LM was 1 × 10−3 M. Deionized water was used in acceptor phase at 298 K. Permeability (P) and flux (J) values for Celgard 2500 and Celgard 2400 membrane are presented in Table 3. Higher porosity of Celgard 2500 offer greater transport efficiency and flux for Cu(II), as compared to Celgard 2400. Thus, in this study Celgard 2500 was selected as the supporting membrane.
Kinetic parameters of Cu(II) for different membrane supports (Carrier = 1 × 10−3 M in diphenyl ether, Cu(NO3)2 = 1 × 10−2 M as donor phase, 298 K and dipping time = 1 h).
Membrane support | P × 106 (m/s1) | J × 105 (mol/m2/s) |
Celgard 2500 | 6.22 ± 0.7 | 1.86 ± 0.08 |
Celgard 2400 | 2.23 ± 0.4 | 0.844 ± 0.01 |
4.5 Effect of accompanying anion on Cu(II) transport
SLM transport also depends on accompanying anion with metal cation. Some typical characteristics of anions that must be accomplished for competent metal transport through SLM are anion lipophilicity, solvent interaction and hydration free energy. Anion interaction and lipophilicity in membrane solvents aid in both co and counter ion-pair mediated transport. Besides, hydration free energy is a determining anion property. Larger anions are easily dehydrated and ready to enter into membrane phase to enhance extraction/complexation rate [39]. Herein, the effect of co-anions on Cu(II) transport was examined by using Cu(NO3)2, CuCl2 and CuSO4 solutions as donor phase individually. The experiment was performed by maintaining as donor phase the 1 × 10−2 M Cu(NO3)2 solution, deionized water as acceptor phase, 1 × 10−3 M 1 in diphenyl ether with Celgard 2500 and 298 K. Permeability (P) and flux (J) values are presented in Table 4. It was inferred from the results that anion lipophilicity is a distinctive property for metal transport through SLM. Owing to the large radius, NO3− and SO42− (0.200 and 0.290 nm, respectively) [40] are easily dehydrated with respect to Cl− (0.168 nm) and has the potential to give better metal transport. But Cu(II) transport is higher with Cl− because in this case, Cl− lipophilicity overcomes its dehydration effect. This fact is in accordance with previously reported work (Table 4) [16,20,21,35,36].
Kinetic parameters of Cu(II) for different co-anions (Carrier = 1 × 10−3 M in diphenyl ether, Celgard 2500, 298 K).
Co-anions | P × 106 (m/s) | J × 105 (mol/m2/s) |
Cu(NO3)2 | 6.22 ± 0.7 | 1.86 ± 0.08 |
CuCl2 | 28.1 ± 0.5 | 3.32 ± 0.05 |
CuSO4 | 5.09 ± 0.4 | 0.788 ± 0.1 |
4.6 Effect of pH of aqueous phases on Cu(II) transport
Another fundamental parameter in metal transport across SLM is the pH of both the aqueous phases. The difference of pH between donor and acceptor phases actually serves as the driving force for metal permeation through LM. The influence of pH on donor phase was investigated by carrying liquid−liquid extraction experiment. The solutions of 1 were extracted with Cu(NO3)2 solutions of pH from 1–6 in order to optimize the pH of the donor phase. HCl was used in making Cu(NO3)2 solutions of varying pH. The changes in the absorption spectra before and after 1 extraction with Cu(NO3)2 solutions were set at different pHs in the range 1–6 are shown in Fig. 5 a and b. The new band after extraction appears at 280 nm that is due to complexation. Maximum complexation relies on several factors, like ionic radii and charge density of metal ion as well as the geometry of both the ligand and metal ion. The detailed explanation about this complexation is published previously [33]. Here, this observation also enlightens the importance of using a macrocylic carrier, i.e. calixarene with distinguishing features, such as aromatic cavity and ease of adding the desired functionality to both its upper and lower rims, which makes it selective for complexation.
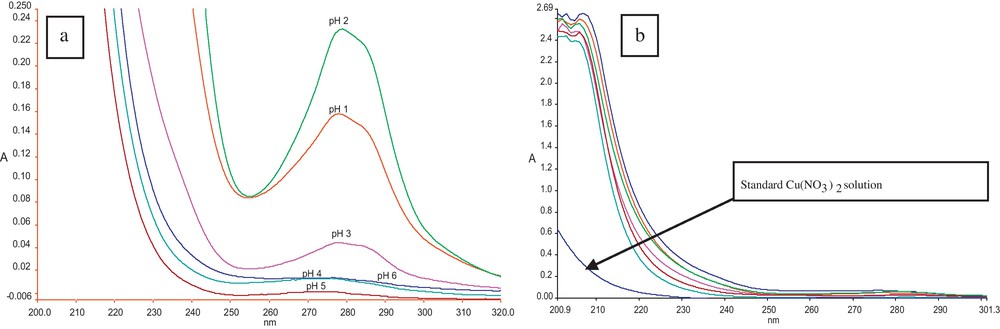
a: Complexation of Cu(NO3)2 from donor phase at pH = 1–6; b: Decomplexation of Cu(NO3)2 into acceptor phase at pH = 5–10. For interpretation of colors, see the online version of this article.
Maximum complexation of 1 with Cu(NO3)2 solution at pH 2 can be justified by considering following equations.
In acidic aqueous solution, Cu(NO3)2 is mostly converted into CuCl2 in presence of HCl.
Cu(NO3)2 + 4HCL → CuCl2 + 2NO + 4H2O + Cl2 |
The carrier 1 is also protonated in organic phase when it comes in contact with acidic aqueous solutions. At pH 2, there is an excess of Cl− ions and more ion pair carrier complexes are in this form [C–Cu2+]·2Cl− rather than [C–Cu2+]·2NO3−. As already discussed in the effect of co-anions, Cl− favors more carrier–Cu(II) complex as compared to NO3−, therefore complextion increases with an increase in the acidity of aqueous solutions. However, low complexation at pH 1 may be due to the competition between proton and Cu(II) for carrier. The formation of crown hydronium ion complex has been reported earlier [41,42].
Acceptor phase pH is also of key importance in the recovery of metal from the membrane phase. In situations where either metal is not completely de-complex at membrane-acceptor interface or it is not prevented from re-entering into the membrane phase, permeation decreases due to saturated membrane phase. Therefore, to check decomplexation efficiency of 1 towards Cu(II) liquid–liquid decomplexation, experiments were performed. Aqueous solutions were prepared in pH range of 5–10 for this study. HCl and NaOH were utilized for pH adjustment accordingly. The graph for decomplexation is presented in Fig. 5b. Acidic and basic media was provided to examine the optimum decomplexation efficiency of p-morpholinomethylcalix[4]arene. But, despite of high absorption, Fig. 5b clearly depicts that decomplexation is the same at all pH; therefore, deionized water is selected for SLM transport. The high absorption may be due to acidic/basic pH and therefore, it is not chosen for further study. In subsequent SLM study, the pH of the donor phase was maintained at 2 and acceptor phase with neutral pH, i.e. pH = 7. The variation in Cu(II) concentration against time is plotted again in Fig. 6 with new pH adjustments of both aqueous phases. The comparison of Fig. 4 and Fig. 6 reveals that more Cu(II) was permeated in less time.

Variation in Cu(II) concentration through supported liquid membrane transport with time. Donor phase: Cu(NO3)2 = 1 × 10−2 M at pH = 2, membrane phase: 1 × 10−3 M carrier in diphenyl ether with Celgard 2500 membrane, acceptor phase: deionized water at 298 K.
4.7 Effect of carrier concentration on Cu(II) transport
The carrier has a profound significance in metal transport through SLM. In carrier mediated transport, carrier serves as a shuttle in bringing metal from donor to membrane phase and subsequent its release into acceptor phase. Thus, the rate of metal permeation increases with a rise in carrier concentration [39].
Thus, to analyze the influence of carrier concentration on metal transport, experiments were performed by varying the carrier concentration in the range 1 × 10−4–1 × 10−2 M. All membrane solutions were prepared in diphenyl ether with Celgard 2500 as support membrane. The concentration of Cu(NO3)2 was 1 × 10−2 M in donor phase at pH = 2 and acceptor phase was deionized water at constant temperature, i.e. 298 K. The results are displayed in Table 5 and Fig. 7. The permeability (P) and flux (J) values increases initially up to 1 × 10−3 M carrier concentration and decreases at a higher concentration. At concentration above 1 × 10−3 M, carrier saturation makes membrane phase more viscous, which results in a low rate of carrier–Cu(II) complexion because viscosity has inverse relation with metal diffusivity. Therefore, 1 × 10−3 M carrier concentration was optimized for Cu(II) transport through SLM.
Kinetic parameters of Cu(II) in different carrier concentrations (Celgard 2500, diphenyl ether, Cu(NO3)2 = 1 × 10−2 M as donor phase at pH = 2, 298 K).
Carrier concentration (M) | P × 106(m/s1) | J × 105 (mol/m2/s) |
1 × 10−4 | 13.2 ± 1.2 | 3.11 ± 0.3 |
5 × 10−4 | 22.4 ± 2 | 4.23 ± 0.5 |
1 × 10−3 | 36.9 ± 1.3 | 13.2 ± 0.7 |
5 × 10−3 | 18.6 ± 1 | 6.21 ± 0.9 |
1 × 10−2 | 7.54 ± 1.5 | 1.67 ± 0.3 |

Effect of carrier concentration on the Cu(II) permeability. Donor phase: Cu(NO3)2 = 1 × 10−2 M at pH = 2, membrane phase: carrier concentration in diphenyl ether with Celgard 2500 membrane, acceptor phase: deionized water at 298 K.
A blank experiment without carrier was also carried out to investigate Cu(II) diffusion in a passive mode. No noticeable Cu(II) transfer was observed in this case, which proves the importance of carrier in facilitated active SLM process.
4.8 Kinetic model
SLM facilitated transport is a combination of two crucial processes, i.e. metal-carrier complexation at both aqueous interfaces and diffusion of complex. Therefore, the overall transport kinetics relies on these two processes. They can be quantified mathematically as D and K. D is the diffusion coefficient of metal–carrier complex which is a measure of diffusion velocity of complex through membrane. The extraction constant, K, is a measure of the ability of carrier to extract metal from the aqueous phase into the membrane phase. Different methods can be applied to determine D, for example flux measurement, determination of lag time (tlag) and Wilke–Chang relation [39,43–46]. In order to investigate which process among diffusion and chemical complexation has dominating influence on metal transport, a dimensionless number α is determined. For α value less than 1, the transport is mainly governed by diffusion of complex while transport is under the influence of chemical complextion for a value greater than 1.
In the present study, D is estimated by three different ways. Initially, Reinhoudt et al. model [43,44,46] was used to calculate D and K from the following equation,
It can be concluded that SLM transport, in this case, is limited by diffusion of metal-carrier complex through the membrane.
Secondly, D was calculated from lag time (tlag) [39,43] measurements, involving metal carrier complex by employing the following equation:
A lag time (tlag) is the time the complex takes to diffuse across the membrane. For 1 × 10−3 M carrier concentration and 100 μm as the membrane thickness, the lag time (tlag) was measured to be 7 s. Therefore, D was calculated to be 1.07 × 10−10 m/s. This value is about to equal to that achieved from Reinhoudt's model.
Thirdly, Wilke–Chang relation [45] was utilized to attain D value which is as follows:
4.9 Mechanism
The mechanism of Cu(II) transport using 1 is illustrated in Fig. 8. Carrier solution in diphenyl ether is immiscible in water and similarly Cu(NO3)2 has limited solubility in membrane solution. However, the carrier can selectively combine with Cu(II) and forms carrier–Cu(II) complex at the donor-membrane interface in acidic conditions. This complex is only soluble in membrane phase and is transferred across the membrane down to its concentration gradient. Decomplexation occurs at membrane-acceptor interface and Cu(II) is released into the acceptor phase. Neutral pH prevents back diffusion to the donor phase. As carrier solution is insoluble, it will not easily leach from the membrane pores. This cycle continues until maximum Cu(II) transfer takes place.

Mechanism of the Cu(II) ion pair mediated transport through supported liquid membrane.
4.10 Comparative study
A comparison of kinetic parameters of p-morpholinomethylcalix[4]arene has been made with other Cu(II) selective carriers as given in Table 6. It has been found that the results obtained in this study are comparatively better than most of the studies reported in the literature [49–58].
Comparison of kinetic parameters of p-morpholinomethylcalix[4]arene with other Cu(II) selective carriers.
Carrier | Membrane solvent/diluent | Carrier concentration | Copper salt | Donor phase faciliting medium | Donorphase pH | Membrane support | Acceptor phase pH | Acceptor phase complexatig medium | Pa (m/s−/1) | J (mol/m2/s1) | D (m/s) | Temperature | Reference | |
1 | Dibenzo-18-Crown-6 (DBI 8C6) | Chloroform | 0.001 M | CuNO3 0.01 M | Thiourea 0.1 M | 1 | Celgard 2500 | 7 | Distilled water | 0.52 × 10−6a | –b | 1.69 × 10−11a | –b | [49] |
2 | Salicylaldoxime (Acorga P-50) | Octane | 0.0158 M | CuSO4 · 5H2O 0.127 M | H2SO4 | 2.63 | Celgard 2500 | 2 | H2SO4 | –b | 1.88 × 10−4a | 1.32 × 10−9a | 298 K | [50] |
3 | LIX 984 | n-Heptane | 10% (v/v) | CuSO4 · 5H2O 20 g/m3 | H2SO4 2.3 M | 1 | Gorotex Teflon | 5 | H2SO4 | –b | –b | 5.72 × 10−10 | 303 K | [51] |
4 | Di-(2-ethylhexyl) phosphoric acid (D2EHPA) | n-Decane | 30% (v/v) | CuSO4 · 5H2O 50 mg/L | H2SO4 | 3.5 | Accurel | 1.5 | –b | –b | 8.70 × 10−4a | –b | 298 K | [52] |
5 | Tris(ethylhexyl) phosphate (TEHP) | Lauric acid | 0.55 M | CuNO3 5 × 10−5 M | N-morpholinoethane sulphonic acid (MES) buffer 10−2 M | 6 | Celgard 2500 | 6.4 | Cyclohexanediamine tetraacetic acid (CDTA) 5 × 10−4 M | –b | 1.90 × 10−6a | –b | –b | [53] |
6 | Acorga M5640 | Iberfluid | 20% (v/v) | Cu (II) in HNO3 Media 1.6 × 10−4 M | –b | 2 | Durapore GVHP04700 | –b | H2SO4 180 g/L | 3.70 × 10−5a | 2.30 × 10−7a | 2.0 × 1012a | –b | [54] |
7 | 5-Nonylsalicylaldoxime (Acorga P-50) | n-Octane | 0.0158 M | CuSO4 · 5H2O 0.127 M | –b | 2.63 | Celgard 2400 | 2 | –b | 7.57 × 10−9a | –b | –b | [55] | |
8 | di(2-ethylhexyl)phosporic acid (D2EHPA) | Coconut oil | 12.4 × 10−4 mol/L | CuSO4 · 5H2O 23.6 × 10−4 M | –b | 4 | –b | –b | H2SO4 0.25 M | –b | 7.4 × 10−9 | 10−9 | –b | [56] |
9 | 3-Phenyl-4-benzoylisoxazol-5-one (HPBI) | Chloroform | 10−2 M | 10−2 M | –b | 5.5 | Celgard 2400 | –b | HNO3 10−1 M | –b | 6.98 × 10−5 | –b | 298 K | [57] |
10 | Oxime derivative (MOC-55 TD) | Iberfluid | 0.36 M | CuSO4 · 5H2O 7.9 × 105 M | –b | 2.5 | Durapore GVHP 4700 | –b | H2SO4 180 g/L−1 | 2.82 × 10−4a | –b | 1.2 × 10−12a | –b | [58] |
11 | p-morpholinomethylcalix[4]arene | Diphenyl ether | 10−3 M | Cu(NO3)2 1 × 10−2 M | –b | 2 | Celgard 2500 | 7 | Deionzed water | 3.69 × 10−5 | 1.32 × 10−4 | 1.54 × 10−10 | 298 K | Current Study |
a The units from the respective reference are converted in SI format.
b This information is not provided in the respective reference.
5 Conclusion
The work reported in this paper revealed that Cu(II) transport from aqueous donor phase can be augmented by using p-morpholinomethylcalix[4]arene (1) as a carrier supported on Celgard 2500 and Celgard 2400 membranes as supported liquid membrane.
The transport efficiency of Cu(II) was greater in diphenyl ether as compared to other solvents because of its more viscosity, low volatility and aqueous immiscibility. Owing to more porosity, Celgard 2500 gave higher Cu(II) permeation than Celgard 2400. The dipping time to prepare carrier 1 SLM was 1 h. The Cu(II) flux values was found to be higher with Cl− as co-anion in comparison to NO3–/SO42– due to the small radius and more solubility in membrane phase. Maximum complexation of Cu(II)–carrier was observed at pH 2 of donor phase and it decomplexed in neutral acceptor phase. The permeation efficiency increased with an increase in carrier concentrations up to 10−3 M and decreased at higher concentrations. The membrane transport mechanism is diffusion-controlled. The diffusion coefficients and extraction constant were calculated from membrane thickness variation studies using Reinhoudt's model. Lag time measurements as well as Wilke–Chang relation were also applied to confirm the diffusion coefficients.
In conclusion, SLM using carrier 1 can be utilized for Cu(II) elimination by making some technical advancement for treating the effluents and by keeping the proper set of conditions.
Acknowledgment
National Centre of Excellence in Analytical Chemistry, University of Sindh, Jamshoro and Higher Education Commission Islamabad Pakistan (No Ps4-086/HEC/Sch/2007) are thanked for facilitating this research work. Prof. Dr. Mustafa Ersöz (Selcuk University, Department of Chemistry, Campus, 42031 Konya, Turkey) and AMTech, Islamabad, Pakistan are also thanked for providing the supported liquid membrane cell and Celgard membranes used in the present study.