1 Introduction
From the perspective of green chemistry, environmentally friendly manufacturing of organic compounds has been considerably re-examined in recent years and many excellent methodologies have been devised. Reactions employing natural sources as catalysts are the best example of such type of aspects.
Laccases are copper-containing glycoprotein oxidoreductase enzymes found in plants and fungi [1,2]. They catalyse the oxidation of a broad range of substrates [3–10], such as methoxyphenols, phenols, o-, and p-diphenols, aminophenols, polyphenols, polyamines, and lignin-related molecules, by reducing dioxygen in a four-electron reduction to water. Moreover, laccases frequently exhibit high oxidative selectivity in aqueous solution and provide a unique green chemistry solution for a variety of oxidations. These properties make laccase attractive biocatalysts in organic synthetic chemistry. Recently, studies have investigated the use of laccase in organic synthesis [11–23].
Meldrum's acid is of particular interest, since they are known to have depressant effect on the central nervous system and to possess a low toxicity, like barbiturates [24]. Catechol, by itself, and mono-substituted catechols are active in part against Pseudomonas, Bacillus, but not Penicillium species [25]. Many flavanoids and catechol derivative turned out to be antimicrobial agents [26]. Hence, the fusion of catechol and Meldrum's acid could provide superior biological moiety i.e. 6,7-dihydroxy-2,2-dimethyl-1,3,9-trioxa-fluoren-4-one. Recently, Nematollahi et al. reported the one-pot reaction of Meldrum's acid and catechol via the oxidation of catechol by an electrochemical method [27]. Because enzymatic oxidation very often parallels the chemical and electrochemical oxidation, it was interesting to study the enzymatic oxidation of catechols and hydroquinones in the presence of Meldrum's acid as CH-acidic nucleophile.
The synthesis of compounds with benzofuran moiety or combination of benzofuran and dioxane make it promising in the search for new pharmacologically active substances, as part of our program aimed at developing efficient and environmentally friendly methodologies for the preparation of fine chemicals [28–30]. So, herein we are studying the enzymatic greener oxidation of catechol/hydroquinone and their derivative in the presence of Meldrum's acid as a nucleophile.
As clearly stated by Sheldon, it is generally recognized that “the best solvent is no solvent and if solvent is needed it should preferably be water”, hence, herein we are using water as the reaction medium. The use of water as the reaction medium represents a remarkable benefit, since this green solvent is highly polar and therefore immiscible with most of the organic compounds; moreover, the water-soluble catalyst resides and operates in the aqueous phase, thus, separation of the organic material is easy [31].
2 Experimental
2.1 Experimental procedures
Meldrum's acid, various catechols and hydroquinones were purchased from Aldrich. Guaiacol was purchased from Hi Media Laboratories Pvt. Ltd. (Mumbai, India). Q-sepharose was purchased from Sigma (St. Louis, USA). All media components and chemicals used were of analytical grade. All reactions and purity of products were monitored by thin layer chromatography (TLC) using aluminium plates coated with silica gel (Merck) employing ethylacetate and hexane (3:7). The isolated products were further purified by column chromatography using aluminium oxide (Sigma–Aldrich 24, 217-9, 70, 35-70, mesh 40 Å, surface area 675 m2/g). IR spectra were recorded on a PerkinElmer FTIR-1710 spectrophotometer using a Nujol film and KBr pellets. 1HNMR, 13CNMR spectra were recorded on a JEOL JNMECX 400P FT NMR system (manufactured in Japan) using TMS as an internal standard. The chemical shift values are recorded on δ scale.
2.1.1 Microorganisms
The laccase produced from Pycnoporous cinnabarinus, were obtained from the Lignocellulose Biotechnology laboratory, University of Delhi, South Campus, New Delhi. The fungal cultures were grown and maintained on malt extract agar (MEA) containing (g/L): malt extract 20.0; KH2PO4 0.5; MgSO4·7H2O, Ca(NO3)2·4H2O 0.5, agar 20.0 (pH 5.4) at 30 °C. Pure cultures were stored at 4 °C and subcultured every fortnight.
2.1.2 Isolation of laccase
Laccase production was carried out using P. cinnabarinus under solid-state fermentation conditions. Then, 1000 g of air-dried wheat bran were spread in separately sterilized enamel trays (78 × 51 × 8.1 cm3) to about 1.0 cm thick and were moistened with a mineral salt solution containing (g/L): KH2PO4 0.5; MgSO4·7H2O, Ca(NO3)2·4H2O 0.5, agar 20.0 (pH 5.4) in 1:2 ratio of solid substrate to moisture. The trays were put in autoclavable poly bags and sterilized at 121 °C (15 psi) for 30 min. The substrate was inoculated with 0.4% w/w of fungal pellets of P. Cinnabarinus. The trays were incubated for 168 h at 30 ± 1 °C in a 70% relative humidity chamber. The laccase from the above three organisms was harvested by adding 0.1 mol/L citrate phosphate buffer (pH 5.4) in a 1:5 ratio of solid substrate to buffer. The mixture was homogenized for 30 min at 30 °C, 200 rpm in a New Brunswick Scientific incubator shaker Innova = 40R (USA). The solid biomass residues were separated from the suspensions by filtration through muslin cloth and the filtrate obtained was centrifuged at 10 000 g for 20 min at 4 °C in a Sigma 6 K 15 laboratory centrifugation system (UK). The supernatant obtained was used as the source of crude enzyme preparations.
2.1.3 Purification of laccase
The crude laccase enzyme extract (1000 mL) from P. cinnabarinus was precipitated by adding ammonium sulphate (40–80% cut off) and centrifuged at 10 000 g for 20 min at 4 °C. The precipitates were resuspended in a 50 mM citrate phosphate buffer (pH 5.4) and dialyzed against the same buffer. This partially purified enzyme sample was loaded to an anion exchange Q-Sepharose column (Sigma–Aldrich, St. Louis, MO, USA), equilibrated with a 10 mM Tris–HCl buffer (pH 7.5). The proteins were eluted by NaCl gradient (0–0.5 M dissolved in equilibrating buffer) at a flow rate of 1 mL/min, with each 1 mL fraction. Fractions with laccase activity were pooled and concentrated using 10-kDa filter membrane (Vivaspin, Vivascience, Sartorious group, Stone house, UK) at 4 °C and assayed for laccase activity.
2.1.4 Enzyme assay
Guaiacol was used as a substrate for assaying laccase activity. One unit of laccase was defined as the change in absorbance of 0.01 mL−1 min−1 at 470 nm [32,33].
2.2 General procedure for the reaction of Meldrum's acid and catechols/hydroquinones
Catechol or hydroquinone (1 mM) and Meldrum's acid (2 mM) were taken in 15 mL of phosphate/citrate buffer pH 7 solution. Then, purified laccase (400 U) and 0.01 mmol of HBT were added to the resultant solution and stirred well at 5 °C with a magnetic stirrer. The temperature of the reaction mixture was gradually increased up to room temperature. The progress of the reaction was monitored by TLC examination at intervals of 30 min. Upon completion of the reaction, approximately after 12 h, the reaction mixture was extracted with ethylacetate (3 × 15, i.e. three-time extraction with 15 mL of ethylacetate). The organic layer was dried over anhydrous Na2SO4. The aqueous phase was further used for another run without any pre-treatment. The product was purified by column chromatography using aluminium oxide as a stationary phase and hexane:ethyl acetate (80:20) as a mobile phase. Products were unambiguously characterized by spectral data.
2.3 Procedure to recover laccase
After completion of the reaction, the product was extracted using ethylacetate and dried over anhydrous sodium sulphate. The solvent was evaporated under reduced pressure and water extract was further used for consecutive runs.
2.4 Characterization of the products
2.4.1 Compound 5a: 6,7-dihydroxy-2,2-dimethyl-1,3,9-trioxa-fluoren-4-one
Mp > 300 °C; IR (KBr) 3430, 3448,1715, 1620, 1618 cm−1; 1HNMR, δ(400 MHz, DMSO-d6) 1.75 (s, 3H), 1.78 (s, 3H), 6.87 (s, 1H), 6.91 (s, 1H), 7.90 (s, br), 8.01 (s, br); 13CNMR, δ(100 MHz, DMSO-d6) 25.1, 25.5, 85.01, 101.23, 105.01, 110.10, 125.11, 127.98, 144.56,151.24, 162.34, 170.20. Anal. calcd. for C12H10O6; C, 57.60; H, 4.03; found C, 57.89, H, 3.98; ESI–MS: 251.00 (M + 1).
2.4.2 Compound 5b: 6,7-dihydroxy-2,2,8-trimethyl-1,3,9-trioxa-fluoren-4-one
Mp > 300 °C; IR (KBr) 3427, 3440,1711, 1621, 1610 cm−1; 1HNMR, δ (400 MHz, DMSO-d6) 1.73 (s, 3H), 1.74 (s, 3H), 2.15 (s, 3H), 6.85 (s, 1H), 7.87 (s, br), 8.00 (s, br); 13CNMR, δ (100 MHz, DMSO-d6) 19.01, 24.9, 25.3, 85.00, 104.91, 109.35,112.01, 124.37, 141.14, 145.38,162.70, 170.00. Anal. calcd. for C13H12O6; C, 59.09; H, 4.58; found C, 59.34, H, 4.38; ESI–MS: 265.12 (M + 1).
2.4.3 Compound 5d: 6,7-dihydroxy-2,2-dimethyl-8-methoxy-1,3,9-trioxa-fluoren-4-one
Mp > 300 °C; IR (KBr) 3426, 3442,1710, 1619, 1617, 1225 cm−1; 1HNMR, δ(400 MHz, DMSO-d6) 1.75 (s, 3H), 1.76 (s, 3H), 3.81 (s, 3H), 6.80 (s, 1H), 7.81 (s, br), 8.00 (s, br); 13CNMR, δ(100 MHz, DMSO-d6) 24.7, 25.0, 61.50, 86.23, 105.97, 110.15, 114.78, 125.11, 142.38, 144.14, 163.25, 171.45. Anal. calcd. for C13H12O7; C, 55.72; H, 4.32; found C, 55.82, H, 4.98; ESI–MS: 281.09 (M + 1).
2.4.4 Compound 5f: 8-(tert-butyl)-6,7-dihydroxy-2,2-dimethyl-1,3,9-trioxa-fluoren-4-on
Mp > 300 °C; IR (KBr) 3421, 3423,1709, 1610, 1611 cm−1; 1HNMR, δ(400 MHz, DMSO-d6) 1.30 (s, 9H), 1.66 (s, 3H), 1.68 (s, 3H), 6.54 (s, 1H), 7.92 (s, br), 8.11 (s, br); 13CNMR, δ(100 MHz, DMSO-d6) 22.7, 22.9, 24.7, 31.6, 84.10, 103.27, 109.11, 111.77, 123.97, 141.45, 142.78, 161.05, 169.35. Anal. calcd. for C15H16O6; C, 62.74; H, 5.92; found C, 61.89, H, 5.58; ESI–MS: 307.10 (M + 1).
2.4.5 Compound 4c: 5-(4, 5-Dihydroxy-2-methyl-phenyl)-2, 2-dimethyl-[1,3]-dioxane-4,6-dione
Mp 245–247 °C; IR (KBr) 3430, 3001,1763, 1724,1698, 1610,1526, 1510 cm−1; 1HNMR, δ(400 MHz, DMSO-d6) 1.70 (s, 3H), 1.78 (s, 3H), 2.10 (s, 3H), 4.41 (s, 1H), 6.55 (s, 1H), 6.80 (s,1H), 7.87 (s, br), 8.00 (s, br); 13CNMR, δ(100 MHz, DMSO-d6) 18.01, 23.9, 24.3, 53.40, 106.11, 115.35, 119.70, 125.17, 127.28, 144.14, 144.10, 168.01. Anal. calcd. for C13H14O6; C, 58.64; H, 5.30; found C, 58.66, H, 5.38; ESI–MS: 267.01 (M + 1).
2.4.6 Compound 4e: 5-(4, 5-Dihydroxy-2-methoxy-phenyl)-2, 2-dimethyl-[1,3]-dioxane-4,6-dione:
Mp 240–242 °C; IR (KBr) 3433, 3010,1766, 1729,1700, 1619,1530, 1519, 1220 cm−1; 1H NMR, δ(400 MHz, DMSO-d6) 1.79 (s, 3H), 1.80 (s, 3H), 3.81 (s, 3H), 4.40 (s, 1H), 6.75 (s, 1H), 6.87 (s,1H), 7.90 (s, br), 8.12 (s, br); 13CNMR, δ(100 MHz, DMSO-d6) 24.1, 24.8, 28.11, 53.55, 107.19, 116.30, 120.61, 124.19, 127.29, 145.19, 147.11, 169.34. Anal. calcd. for C13H14O7; C, 55.32; H, 5.00; found C, 55.39, H, 4.98; ESI–MS: 283.02 (M + 1).
2.4.7 Compound 4g: 5-(2-(tert-butyl)-4, 5-Dihydroxyphenyl)-2,2-dimethyl-1,3-dioxane-4,6-dione
Mp 215–217 °C; IR (KBr) 3430, 3009,1761, 1727,1696, 1613,1518, 1512 cm−1; 1HNMR, δ(400 MHz, DMSO-d6) 1.35 (s, 9H), 1.70 (s, 3H), 1.72 (s, 3H), 4.38 (s, 1H), 6.72 (s, 1H), 6.86 (s,1H), 7.78 (s, br), 8.10 (s, br); 13CNMR, δ(100 MHz, DMSO-d6) 27.1, 27.3, 31.13, 34.25, 53.55, 107.19, 116.30, 120.61, 124.19, 127.29, 145.19, 147.11, 169.34. Anal. calcd. for C16H20O6; C, 62.33; H, 6.54; found C, 62.99, H, 6.98; ESI–MS: 309.11 (M + 1).
2.4.8 Compound 9a: 5,8-Dihydroxy-2,2-dimethyl-1,3,9-trioxa-fluoren-4-one
Mp > 300 °C; IR (KBr) 3433, 3449, 1712, 1620, 1618 cm−1; 1HNMR, δ(400 MHz, DMSO-d6) 1.70 (s, 3H), 1.77 (s, 3H), 6.84 (d, 1H), 6.89 (d, 1H), 8.00 (s, br), 8.11 (s, br); 13CNMR, δ(100 MHz, DMSO-d6) 24.4, 25.5, 80.01, 100.23, 104.11, 110.19, 124.11, 128.00, 144.60,150.94, 162.14, 170.00. Anal. calcd. for C12H10O6; C, 57.60; H, 4.03; found C, 57.67, H, 4.17; ESI–MS: 251.34 (M + 1).
2.4.9 Compound 9b: 5,8-Dihydroxy-2,2,7-trimethyl-1,3,9-trioxa-fluoren-4-one
Mp > 300 °C; IR (KBr) 3425, 3442,1710, 1619, 1605 cm−1; 1HNMR, δ(400 MHz, DMSO-d6) 1.73 (s, 3H), 1.76 (s, 3H), 2.10 (s, 3H), 6.90 (s, 1H), 7.80 (s, br), 8.04 (s, br); 13CNMR, δ(100 MHz, DMSO-d6) 19.00, 25.02, 25.37, 86.12, 104.91, 109.40, 112.34, 125.17, 141.10, 145.38,161.99, 171.14. Anal. calcd. for C13H12O6; C, 59.09; H, 4.58; found C, 59.24, H, 4.40; ESI–MS: 265.54 (M + 1).
2.4.10 Compound 9d: 5,8-Dihydroxy-2,2-dimethyl-7-methoxy-1,3,9-trioxa-fluoren-4-one
Mp > 300 °C; IR (KBr) 3424, 3440,1710, 1620, 1617, 1224 cm−1; 1HNMR, δ(400 MHz, DMSO-d6) 1.73 (s, 3H), 1.75 (s, 3H), 3.80 (s, 3H), 6.79 (s, 1H), 7.92 (s, br), 7.99 (s, br); 13CNMR, δ (100 MHz, DMSO-d6) 24.74, 25.50, 63.00, 88.13, 104.17, 108.34, 114.78, 125.71, 142.89, 146.24, 165.05, 172.95. Anal. calcd. for C13H12O7; C, 55.72; H, 4.32; found C, 56.02, H, 4.18; ESI–MS: 280.99 (M + 1).
2.4.11 Compound 9f: 7-(tert-butyl)-5,8-dihydroxy-2,2-dimethyl-1,3,9-trioxa-fluoren-4-one
Mp > 300 °C; IR (KBr) 3423, 3422,1706, 1615, 1618 cm−1; 1HNMR, δ (400 MHz, DMSO-d6) 1.28 (s, 9H), 1.64 (s, 3H), 1.66 (s, 3H), 6.50 (s, 1H), 8.02 (s, br), 8.16 (s, br); 13CNMR, δ (100 MHz, DMSO-d6) 21.90, 22.84, 23.17, 31.65, 85.18, 102.17, 108.00, 110.79, 124.40, 143.25, 144.78, 163.25, 170.16. Anal. calcd. for C15H16O6; C, 62.74; H, 5.92; found C, 62.80, H, 5.98; ESI–MS: 307.28 (M + 1).
2.4.12 Compound 8c: 5-(3,6-Dihydroxy-2,4-dimethyl-phenyl)-2,2-dimethyl-[1,3]-dioxane-4,6-dione
Mp > 300 °C; IR (KBr) 3427, 3006, 1766, 1728,1690, 1609,1528 cm−1; 1HNMR, δ(400 MHz, DMSO-d6) 1.69 (s, 3H), 1.76 (s, 3H), 2.09 (s, 3H), 2.15 (s, 3H), 4.38 (s, 1H), 6.77 (s,1H), 7.80 (s, br), 8.07 (s, br); 13CNMR, δ (100 MHz, DMSO-d6) 17.91, 23.98, 24.97, 55.20, 59.24, 106.11, 115.35, 119.70, 125.17, 127.28, 140.14, 143.17, 167.00. Anal. calcd. for C13H14O6; C, 59.99; H, 5.75; found C, 59.66, H, 5.78; ESI–MS: 280.22 (M + 1).
2.4.13 Compound 8e: 5-(3,6-Dihydroxy-2,2-dimethoxy-phenyl)-2,2-dimethyl-[1,3]-dioxane-4,6-dione
Mp > 300 °C; IR (KBr) 3430, 3009,1772, 1730,1704, 1610,1533 cm−1; 1HNMR, δ (400 MHz, DMSO-d6) 1.76 (s, 3H), 1.78 (s, 3H), 3.80 (s, 3H), 4.40 (s, 1H), 6.87 (s,1H), 7.89 (s, br), 8.10 (s, br); 13CNMR, δ (100 MHz, DMSO-d6) 24.1, 24.8, 28.11, 53.55, 107.19, 116.30, 120.61, 124.19, 127.29, 145.19, 147.11, 169.34. Anal. calcd. for C13H14O7; C, 55.32; H, 5.00; found C, 55.39, H, 4.98; ESI–MS: 283.02 (M + 1).
3 Results and discussion
To explore the catalytic activity of laccase for the coupling of catechol and Meldrum's acid, a trial reaction was performed using 1.0 mM catechol in aqueous solution containing 0.1 M acetate buffer, (pH = 5.0), 2.0 mM Meldrum's acid and 400 U of laccase. 6,7-Dihydroxy-2,2-dimethyl-1,3,9-trioxa-fluoren-4-one was obtained in 45% yield when the reaction was stirred for 10 h. The same reaction was performed in the absence of laccase; no yield of product was formed, as catechol is unable to be converted into o-quinone in the absence of laccase. An excess of Meldrum's acid was required to overcome the intrinsic instability of the o-benzoquinones as it will undergo competing decomposition, dimerization and polymerization if insufficient amounts of dione are present for the Michael addition. The low pKa value of Meldrum's acid [34,35] allowed the use of neutral conditions (acetate buffer pH 7) for the enzymatic study of catechols in the presence of Meldrum's acid. Hence, the same reaction was tried at pH 7. This resulted in an increase in the yield of the coupling product (55%). To further explore the catalyst divergence, the same reaction was carried out with 1,4-benzoquinone in the place of catechol. It was found that 1,4-benzoquinone is as reactive as catechol and gives the desired product in quantitative yield (Schemes 1 and 2).

Synthesis of 6,7-dihydroxy-2,2-dimethyl-1,3,9-trioxa-fluoren-4-one using catechol and Meldrum's acid at room temperature, using laccase as a catalyst in phosphate/citrate buffer at pH 7.

Synthesis of 5,8-dihydroxy-2,2-dimethyl-1,3,9-trioxa-fluoren-4-one using hydroquinone and Meldrum's acid, using laccase as a catalyst in phosphate/citrate buffer at pH 7.
In addition, the reaction temperature was shown to have an effect on the reaction outcome. For example, if the reaction was performed at room temperature or 60 °C, the yield of the product was diminished by only 0–10%. This result was attributed to an increase in the rate of decomposition and polymerization of the in situ generated o-quinone and of in situ decomposition of Meldrum's acid–catechol derivative to benzofuran as a side-product because of the prone tendency of Meldrum's acid to decompose into CO2 and acetone. Therefore, we retarded the rate of decomposition and polymerization by maintaining the initial temperature to 5 °C for first 2 h and then, allowing the reaction mixture to come up to room temperature.
The enzymes have been immobilized using several methodologies for their biocatalytic applicability affecting their activity, effectiveness of utilization, deactivation, regeneration kinetics and cost [36], without considering the toxicity of the immobilized reagents and the waste disposal problems. Hence, in this study, the laccase-catalysed synthesis was carried out in aqueous medium for three consecutive runs and monitored by TLC. Table 1 depicts the yield of the product from the reaction catalysed by laccase in three consecutive runs. The yield was observed to decrease slightly after each run.
Catalyst recycling studiesa.
Catalyst recycle | Time (h) | Yieldb (%) |
1 | 12 | 55 |
2 | 12 | 52 |
3 | 12 | 49 |
a Reaction conditions: 1 mmol of 1 (Meldrum's acid) and 2 mmol of 3 (catechol) were taken in 15 mL of buffer solution and 400 U of laccase and stirred well for 12 h.
b Isolated yield.
Table 2 shows the effect of different solvent systems on the product yield. In DMSO, DMF, and MeCN, no reaction was observed due to the denaturation of laccase. The reaction in aqueous buffer proceeded in higher yield using buffer tablets of NaH2PO4, Na2HPO4, and potassium phthalate (pH 7). The lower percentage yields in other solvent systems were due to a decrease in laccase activity in non-intermiscible organic and aqueous phases. Moreover, the domino reaction was shown to exhibit higher reactivity and selectivity in aqueous medium rather than in organic solvents.
Solvent optimization for 1,3,9-trioxa-fluoren-4-one derivativesa.
S. No. | Solvent | Yieldb (%) |
1 | 1 M acetate buffer pH 4 | 23 |
2 | Water | 28 |
3 | THF | 31 |
4 | Ethanol | 34 |
5 | 1 M phosphate/citrate buffer pH 4.5 | 45 |
6 | 1 M phosphate/citrate buffer pH 7 | 55 |
7 | DMSO | – |
8 | MeCN | – |
9 | DMF | – |
10 | Methanol | 30 |
11 | 1,4-Dioxane | 18 |
a Reaction conditions: 1 mmol 1 (Meldrum's acid) and 2 mmol of 3 (catechol) were taken in 15 mL of solvent and 400 U of laccase (P. cinnabarinus) were added and the resultant solution was stirred well for 12 h.
b Isolated yield.
3.1 Effect of enzyme concentration
The effect of enzyme concentration on the reaction was studied to determine the minimum amount of enzyme required for a maximum formation of desired product. It is essential to optimize the concentration of the enzyme in the reaction mixture because it greatly influences the reaction rate. In the present investigation, it was observed that the rate of laccase-catalysed product formation increased with increasing enzyme concentration and decreased after reaching optimal enzyme concentration. A similar trend was observed for all the derivatives. About 400 U of laccase is used as a standard concentration for all the reactions (Table 3).
Synthesis of 1,3,9-trioxa-fluoren-4-one derivatives using catechol and Meldrum's acid, with different concentration of laccase (P. cinnabarinus) in phosphate/citrate buffer at pH 7a
S. No. | Concentration of enzyme (U) | Yieldb (%) |
1 | 100 | 21 |
2 | 200 | 30 |
3 | 400 | 55 |
4 | 500 | 57 |
a Reaction conditions: 1 mmol 1 (Meldrum's acid) and 2 mmol of 3 (catechol) were taken in 15 mL of buffer solution. Different units of laccase were added and the resultant solution was stirred well for 12 h.
b Isolated yield.
3.2 Effect of redox mediator on product formation
When laccase was used as the sole catalyst, the yield of the product was very less. Hence, synthetic redox mediators, 1-hydroxybenzotriazole (HBT), N-hydroxyphthalimide (HPI) and 2,2-azinobis (3-ethylbenzthiazoline-6-sulfonate) (ABTS) were employed along with laccase as laccase mediator systems (LMSs) for improvisation of the product (Fig. 1).
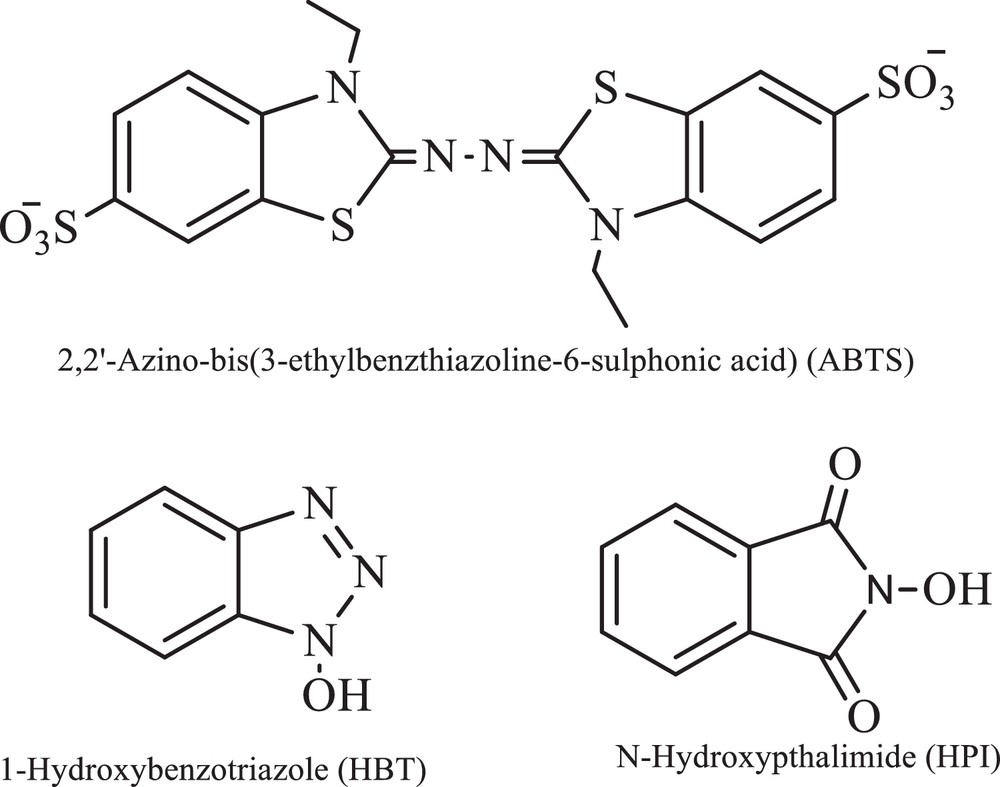
Various mediators used.
The mediator needs to be easily oxidised by laccase to the Medox status (Scheme 3).
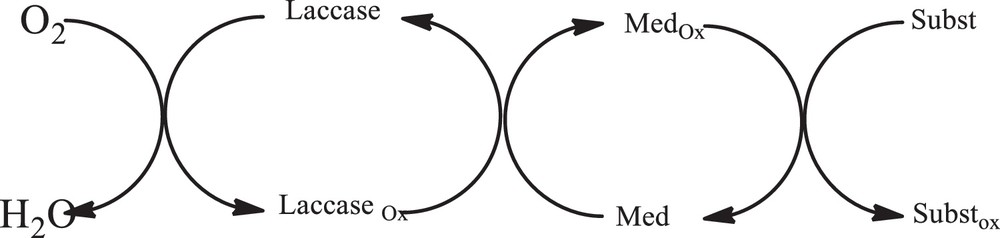
General mechanism for the reaction between different catechols and Meldrum's acid in the presence of laccase.
1-Hydroxybenzotriazole [37] (HBT) and N-hydroxyphthalimide [38] (HPI) are two N–OH compounds that are known to mediate the activity of laccase, driving it towards non-phenolic substrates, and the relative efficiency of these mediators has been recently evaluated [39]. A consensus has been reached upon the preliminary mono-electronic oxidation of these N–OH mediators by laccase to the corresponding N-oxyl radical (N–O•) forms [40] through the fleeting intervention of their radical cations, HPT•+ or HPI•+, respectively. Two different hypotheses have been proposed to explain the subsequent oxidation of the substrate. Either these N–O• radicals perform a one-electron oxidation (ET) of the substrate to a radical cation [41], or they abstract the H-atom from the substrate (HAT), converting it into a radical [42]; this supports the ET route with laccase/ABTS through the likely formation of ABTS++. All the mediators used enhanced the rate of reaction appreciably but not enough to make the reaction economically viable; HBT was used as a mediator. (Table 4).
Synthesis of 1,3,9-trioxa-fluoren-4-one derivatives using different catechol and Meldrum's acid in laccase (P. cinnabarinus) using different mediators in phosphate/citrate buffer at pH 7a.
S. No. | Different mediators used | Yieldb (%) |
1 | HPI | 59 |
2 | ABTS | 65 |
3 | HBT | 65 |
a Reaction conditions: 1 mmol 1 (Meldrum's acid) and 2 mmol of 3 (catechol) were taken in 15 mL of buffer solution and 100 U of laccase (P. cinnabarinus) with different mediators was added and the resultant solution was stirred well for 12 h.
b Isolated yield.
After developing the optimum reaction conditions, the reaction of a variety of catechol and hydroquinone derivatives were examined and these results are explained comprehensively (Tables 5 and 6).
Synthesis of 1,3,9-trioxa-fluoren-4-one derivatives using different catechol and Meldrum's acid employing 400 U of laccase (P. cinnabarinus) in phosphate/citrate buffer at pH 7a.
S. No. | Product | R1 | R2 | Yield (%)b | Time(h) |
1 | 5a | H | H | 65 | 12 |
2 | 5b | –CH3 | H | 61 | 12 |
3 | 4c | H | –CH3 | 35 | 12 |
4 | 5d | –OCH3 | H | 51 | 12 |
5 | 4e | H | –OCH3 | 40 | 12 |
6 | 5f | –C(CH3)3 | H | 39 | 12 |
7 | 4g | H | –C(CH3)3 | 39 | 12 |
8 | 5h | H | F | – | 12 |
9 | 4i | F | H | – | 12 |
a Reaction conditions: 1 mmol 1(a–i) and 2 mmol of 3 (Meldrum's acid) were taken in 15 mL of solvent and 400 U of laccase and 0.01 mmol of HBT were added and the resultant solution was stirred well for 12 h.
b Isolated yield.
Synthesis of 1,3,9-trioxa-fluoren-4-one derivatives using different hydroquinones and Meldrum's acid employing 400 U of laccase (P. cinnabarinus) phosphate/citrate buffer of pH 7a.
S. No. | Product | R1 | R2 | Yield (%)b | Time (h) |
1 | 9a | H | H | 68 | 12 |
2 | 9b | –CH3 | H | 61 | 12 |
3 | 8c | –CH3 | –CH3 | 40 | 12 |
4 | 9d | –OCH3 | H | 59 | 12 |
5 | 8e | –OCH3 | –OCH3 | 41 | 12 |
6 | 9f | –C(CH3)3 | H | 43 | 12 |
7 | 8g | –C(CH3)3 | –C(CH3)3 | – | 12 |
8 | 9h | F | H | – | 12 |
a Reaction conditions: 1 mmol 6(a–h) and 2 mmol of 3 (Meldrum's acid) were taken in 15 mL of solvent and 400 U of laccase and 0.01 mmol of HBT were added and the resultant solution was stirred well for 12 h.
b Isolated yield.
The rate of reaction and the nature of the products are dependent on the nature and position of the substituted group on the catechol ring. The presence of bulky groups, such as tert-butyl, on the catechol ring causes a decrease in rate of the reaction. Furthermore, 3-fluoro-1,2-benzoquinone, which has a strong electron withdrawing group (F), did not provide any desired product. This reactivity pattern may be caused by side reactions of highly reactive quinone.
Besides 3-substituted catechols, 4-substituted catechols, such as 4-methyl/4-chlorocatechol or 3,4-dihydroxybenzoic acid were also used for the reaction, but the presence of substituted groups in the C-4 position of the catechol ring, which is a reactive site of o-benzoquinones, causes a decrease in the rate of reaction and stops the reaction at a particular stage and does not lead to the final desired product. It was found that the reaction stopped with the formation of product 4 in which the catechol ring is already occupied with a substituent and does not permit the formation of the desired cyclised product. A general reaction mechanism is demonstrated in Scheme 4 and shows the formation of products 4 and 5 under different circumstances.

General mechanism for the reaction between different catechol and Meldrum's acid in the presence of laccase.
It was found that when 3-substituted catechols were used with Meldrum's acid, then approximately 65% of desired product (5) was separated out. Side-products may contain partially reacted product (4), but in too small amounts to be separated. When 4-substituted catechols were used with Meldrum's acid, then, there is no scope for the formation of the desired product (5). The only separated product was (4).
1H-NMR of catechol gave a multiplet for aromatic hydrogens in the 6.82–6.94 ppm region; Meldrum's acid gave a singlet in the 3.10–2.30 ppm region for methylene protons and two singlets at around 1.59 and 1.61 for methyl group protons, respectively. On the other hand, our product (5) displays no multiplet in the aromatic region, which confirms that two of the aromatic protons are removed during the coupling with Meldrum's acid. The peak for the methylene protons of Meldrum's acid has also disappeared. Moreover, the ppm values for the methyl proton of Meldrum's acid are changed as it is coupled with the catechol ring. In the case of product (4), it was observed that the peak at approximately 4.4 is due to the methine proton left on Meldrum's acid, as it does not participate in the reaction due to the occupancy of the C-4 position of catechol.
When substituted hydroquinone was used in place of substituted catechol, the same pattern was observed. But when 2,6-disubstituted hydroquinone was used, then a decrease in the rate of reaction was observed and the reaction stops at a particular stage, and does not lead to the desired final product (Scheme 5).

General mechanism for the reaction between different hydroquinones and Meldrum's acid in the presence of laccase.
4 Conclusion
The laccase-catalysed reaction for the coupling of catechols/hydroquinones and Meldrum's acid has been scrutinized with a view to the synthesis of new series of compounds. This investigation is tunable for catalyst and solvent to facilitate the reaction and the reduction of waste, with the use of more benign solvents. Hence, in addition to the simple reaction conditions, the easy work-up procedure makes our methodology a valid contribution to the existing processes in the field of enzymatic catalysis.
Acknowledgements
The authors (M. Kidwai and A. Jain) are thankful to UGC New Delhi for providing financial assistance. The authors (A. Sharma and R.C. Kuhad) acknowledge the financial support from DBT.