1 Introduction
Hydantoins, 2,4-imidazolidine-diones, are a familiar five-membered heterocycles including a useful reactive urea moiety [1,2]. Various hydantoin-containing natural and synthetic products show different biological activities, such as anti-ulcer [3], anti-tumor [4], antiviral [5], antimicrobial [6], anti-diabetic [7], anti-arrhythmic [8], anticonvulsant [9] properties; they can also be used for agrochemical (herbicidal and fungicidal) applications [10]. Several methods are used for the preparation of hydantoins [11–15]. 5,5-Disubstituted hydantoins are often made by Bucherer–Bergs synthesis and Reed syntheses [16,17]. However, some of the reported methods suffer from drawbacks, such as poor yields, long reaction times, expensive substrates, difficult reaction conditions, complexity of work-up and co-occurrence of several side reactions, as well as environmental concern. Therefore, the development of simple, high-yielding and eco-friendly methods using new catalysts for the preparation of hydantoin derivatives would be highly desirable.
Recently, iron oxide magnetic nanoparticles (Fe3O4 MNPs) have gained increasing interest in many areas, due to their properties, including super paramagnetic activities, high surface area, low toxicity, and simple separation by an external magnetic field [18–20]. Magnetic particles are used in different fields, such as medicinal applications, drug delivery, and industry [21]. Additionally, Fe3O4 nanoparticles (nano-Fe3O4) have emerged as a valuable group of heterogeneous catalysts due to their application in nanocatalysis. Fe3O4 nanoparticles are economic nanocatalysts and are environmentally benign. Moreover, they can be easily separated and recycled from the products by an external magnet [22]. During the past decades, magnetic nanocatalysts were used in several organic reactions, including the synthesis of α-aminonitriles [23], the coupling of phenols with aryl halides [24], the Suzuki reaction [25], the synthesis of propargylic amines [26], the synthesis of sulfonamides [27], the synthesis of quinoxalines [28], the Sonogashira–Hagihara coupling reaction [29], the aza–Michael addition, and the Paal–Knorr reaction [30].
In this study, high activation and regeneration of Fe3O4 nanoparticles as heterogeneous catalyst in the synthesis of hydantoins are shown. The use of MNPs not only gives high yields in short reaction times, but it is also a cheap and facile method.
Herein, we wish to report a simple and one-pot procedure for the synthesis of 5,5-disubstituted hydantoins using magnetic Fe3O4 nanoparticle as a magnetic catalyst (Scheme 1).

Synthesis of hydantoin derivatives by using magnetic Fe3O4 nanoparticles under solvent-free conditions.
2 Results and discussion
In this work, we prepared hydantoin derivatives using the Bucherer–Bergs reaction in the presence of Fe3O4 nanoparticles as a solid catalyst under solvent-free conditions. Firstly, Fe3O4 nanoparticles were prepared by chemical co-precipitation with a size range of 18 nm. Fig. 1 shows the XRD patterns of the Fe3O4 nanoparticles.

XRD patterns of Fe3O4 MNPs.
The XRD patterns of the particles show six characteristic peaks reveal a cubic iron oxide phrase (2h = 30.35, 35.95, 43.45, 53.70, 57.25, 62.88, 71.37, 74.46). These are related to their corresponding indices (220), (311), (400), (331), (422), (333), (440) and (531), respectively. It implies that the resultant nanoparticles are pure Fe3O4 with a spinel structure. The crystal size of MNPs can be determined from the XRD pattern by using the Debye–Scherrer equation:
D(hkl) is the average crystalline diameter, 0.94 is the Scherrer constant, λ is the X-ray wavelength, β is the half-width of XRD diffraction lines and θ is the Bragg angle, in degree. The (311) peak of the maximum intensity was picked out to estimate the nanoparticles’ diameter. The XRD patterns of maghemite and magnetite are very close. Thus, the lattice parameter must be precisely determined. Magnetite is an inverse spinel with a lattice parameter of 8.3941 Å. Cations are arranged with one Fe3+ per filled tetrahedral hole, and the remaining Fe3+ and Fe2+ are distributed in the octahedral holes.
The lattice parameter of the synthesized magnetite is 8.3850 Å, which is consistent with the expected lattice parameter for magnetic Fe3O4 particles. This value is closer to that of stoichiometric magnetite (8.3941 Å) than to that of maghemite (8.346 Å) [31,32].
A SEM image is shown in Fig. 2a. The SEM image shows that magnetite nanoparticles have a mean diameter of about 18 nm and a nearly spherical shape, which is consistent with XRD results. The SEM image shows the shape and size of the nanoparticles. Also, transmission electron microscopy (TEM) analyses were used for characterization. The TEM image discloses the spherical Fe3O4 particles with an average size of about 18 nm (Fig. 2b).

SEM (a) and TEM (b) images of MNPs.
Fig. 3 shows the Fourier transform infrared (FT–IR) spectra of MNPs. The absorbance band at 580 cm−1 is ascribed to the Fe–O stretching vibration, which is consistent with the reported IR spectra for spinel Fe3O4 [33]. The difference between the spectra of maghemite and magnetite is related to the absence of Fe2+ cations, and the presence of vacancies within the OH sites. It should be noted that the IR spectrum of Fe2O3 (maghemite) reported in the literature is more complicated than that of Fe3O4 (magnetite) [34–36].
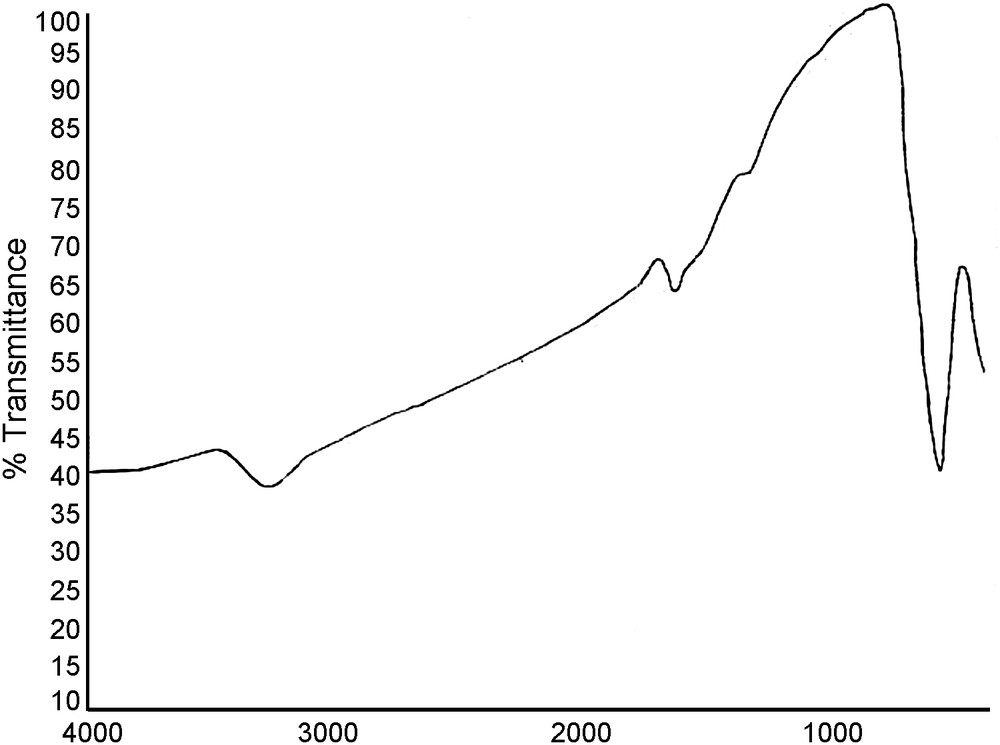
FT–IR spectra of the Fe3O4 MNPs.
In continuation of our research to optimize the reaction conditions, the synthesis of 5-phenylhydantoin as a model reaction was chosen for the synthesis of 5,5-disubstituted hydantoins. We examined the model reaction in the presence of an optimized molar ratio of nanocatalyst (1 mol%), aldehyde or ketone (1 mmol), potassium cyanide (1.3 mmol), and ammonium carbonate (6 mmol) in different solvents, such as ethanol, methanol, DMSO, CH3CN, ethanol:H2O (ratio of 1:1) and solvent-free conditions at 70 °C. As shown in Table 1, solvent-free conditions are the best ones for the synthesis of hydantoins.
Screening of the effect of the solvent on the model reactiona.
Entry | Solvent | Time (min) | Yield (%)b |
1 | Ethanol | 20 | 80 |
2 | Methanol | 20 | 70 |
3 | Methanol:H2O | 17 | 80 |
4 | DMSO | 20 | 50 |
5 | CH3CN | 20 | 48 |
6 | Ethanol:H2O | 17 | 90 |
7 | Solvent-free | 15 | 93 |
a Catalyst amount (1 mol%) under solvent-free conditions.
b Isolated yield of the pure compound.
After this, the reaction was carried out both in the presence and in the absence of the Fe3O4 nanocatalyst. We evaluated the model reaction in the presence of 0.1, 0.5, 1.0, 2.0 and 5.0 mol% of MNPs to find out the effect of the amount of catalyst. As indicated in Table 2, the maximum yield (95%) was obtained when 1 mol% of nanocatalyst was used. Increasing this amount of nano-Fe3O4 did not cause any increase of the yield.
Screening of the effect of molar ratio on the model reaction.
Entry | Catalyst (mol%) | Time (min) | Yield (%)a |
1 | 0.0 | 30 | 20 |
2 | 0.1 | 20 | 75 |
3 | 0.5 | 15 | 91 |
4 | 1.0 | 15 | 95 |
5 | 3.0 | 15 | 95 |
6 | 5.0 | 15 | 95 |
a Under solvent-free condition at 70 °C.
Also, in order to further improve the conditions of the reaction, we investigated the model reaction in solvent-free conditions and with 1 mol% of MNPs at room temperature, 40, 50, 70, 90, and 110 °C to find out the effect of the temperature on the progress of the reaction. It was observed that the higher reaction temperature gave the higher yield. As shown in Table 3, the optimum temperature for the reaction was 70 °C.
Screening of the effect of temperature on the model reaction.
Entry | Temperature (°C) | Yield (%) | Time (min) |
1 | RT | 27 | 30 |
2 | 40 | 80 | 20 |
3 | 50 | 87 | 15 |
4 | 70 | 95 | 15 |
5 | 90 | 95 | 15 |
6 | 110 | 95 | 15 |
Therefore, we selected magnetic Fe3O4 nanoparticles as an efficient catalyst for the synthesis of hydantoin derivatives at 70 °C under solvent-free conditions.
Next, we performed the synthesis of diverse 5,5-disubstituted hydantoins with different substituted carbonyl compounds (aldehyde or ketone) under optimized reaction conditions in excellent yields (Table 4). The carbonyl compounds containing electron-withdrawing groups on the aromatic ring were found to be likely more reactive and could react with ammonium carbonate rapidly. In contrast, the ketones (or aldehydes) containing electron-donating groups on the aromatic ring have shown lower reactivity.
One-pot synthesis of 5,5-disubstituted hydantoins catalyzed by Fe3O4 nanoparticles under solvent-free conditions at 70 °Ca.
Entry | R1 | R2 | Productb | Time (min)/Yield (%)c | Mprep/Mplit (°C) |
1 | H | C6H5 | 4a | 17/95 | (164–165)/(163) [37] |
2 | H | 4-CH3C6H5 | 4b | 19/88 | (183–184)/(182.5) [38] |
3 | H | 4-OCH3C6H5 | 4c | 23/95 | (193–197)/– |
4 | H | 2-OCH3C6H5 | 4d | 31/88 | (183–185)/(186–187) [38] |
5 | H | 2-ClC6H5 | 4e | 20/95 | (178–179)/(176) [38] |
6 | H | n-Bu | 4f | 14/97 | (142–143)/(139.5) [38] |
7 | H | i-Bu | 4g | 17/95 | (214–217)/(212.5–216) [38] |
8 | CH3 | i-Bu | 4h | 25/98 | (148–150)/(148) [38] |
9 | 4i | 25/99 | (219–221)/(220) [37] | ||
10 | CH3 | C6H5 | 4j | 30/88 | (197–199)/(195–196) [39] |
11 | CH3 | 4-ClC6H4 | 4k | 30/95 | (261–262)/(262–263) [40] |
12 | CH3 | 3-CH3C6H4 | 4l | 33/97 | (180–182)/(175–180) [39] |
13 | CH3 | 3-ClC6H4 | 4m | 27/96 | (180–183)/(180–182) [39] |
14 | C6H5 | C6H5 | 4n | 54/85 | (295–296)/(297–298) [41] |
15 | 4o | 60/81 | (323–326)/(324–325) [38] |
a Aldehyde or ketone (1 mmol), KCN (1.3 mmol), (NH4)2CO3 (6 mmol), Fe3O4 MNPs (1 mol%)
b All the products were characterized by IR, 1H NMR and 13C NMR spectra.
c Isolated yields.
At the end of the reaction, the magnetic Fe3O4 nanoparticles were washed with diethylether and dried at 130 °C for 4 h. Also, the reusability of the MNPs was surveyed. We found that after reusing the MNPs four times, no change was observed in their catalytic activity.
Moreover, a plausible mechanism for the formation of hydantoin derivatives is shown in Scheme 2. Fe3O4 nanoparticles as a Lewis acid can activate the carbonyl group of the ketone (or the aldehyde) (1) to increase the electrophilic character of the carbonyl groups and decrease the energy of the transition state [33]. This was followed by the attack of ammonia on the activated carbonyl group, resulting in the formation of imine. The α-aminonitrile compound is prepared via the nucleophilic attack of imine by the cyanide ion in the presence of nano-Fe3O4 as a nanocatalyst. From the condensation of α-aminonitrile with carbon dioxide, hydantoin (4) was produced. In fact, Fe3O4 nanoparticles are required for the formation of both the imine and the α-aminonitrile [42]. Also, a Lewis acid, such as Fe3O4 nanoparticles may assist in the formation of the hydantoin from aminonitrile by the treatment with carbon dioxide [43].
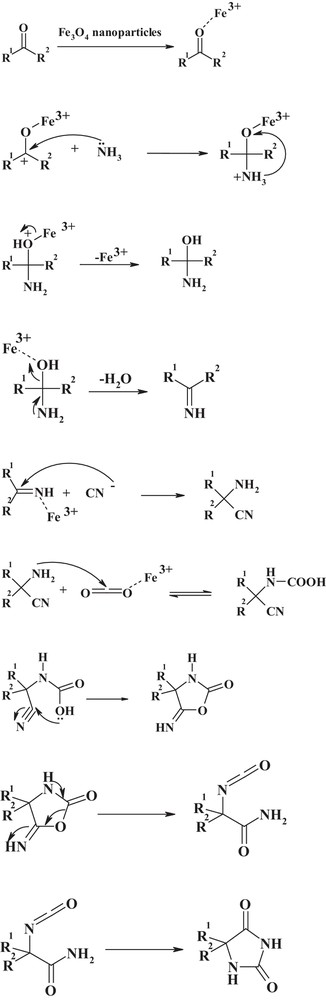
Possible mechanism for the preparation of hydantoin derivatives.
3 Conclusions
In conclusion, we reported an efficient approach for the preparation of 5,5-disubstituted hydantoin derivatives via the one-pot three-component condensation of aldehyde (or ketone), KCN and (NH4)2CO3 in the presence of magnetic Fe3O4 nanoparticles as a safe and inexpensive magnetic catalyst under solvent-free conditions. The separation of the MNPs was very effective, simple and economical. The heterogeneous magnetic catalyst could be reused several times without significant loss of the catalytic performance of MNPs. It is of interest to note that this procedure will find important applications in the synthesis of hydantoin derivatives.
4 Experimental method
4.1 Materials and techniques
Chemical reagents in high purity were purchased from the Merck Chemical Company. All the materials were of commercial reagent grade. Melting points were determined in open capillaries using an Electrothermal Mk3 apparatus and are uncorrected. 1H NMR and 13C NMR spectra were recorded with a Bruker DRX-400 spectrometer at 400 and 100 MHz, respectively. NMR spectra were obtained in DMSO-d6 solutions and are reported as parts per million (ppm) downfield from tetramethylsilane as the internal standard. The abbreviations used are: singlet (s), doublet (d), triplet (t) and multiplet (m). FT–IR spectra were obtained with potassium bromide pellets in the range 400–4000 cm−1 with a PerkinElmer 550 spectrometer. The elemental analyses (C, H, N) were obtained with both Carlo Erba Model EA 1108 and PerkinElmer 240c analyzers. The UV–vis measurements were obtained with a GBC cintra 6 UV–vis spectrophotometer. The melting points were obtained with a micro melting point apparatus (Electrothermal, MK3) and are uncorrected. Nanostructures were characterized using a Holland Philips Xpert X-ray powder diffraction (XRD) diffractometer (Cu Kα, radiation, λ = 0.154056 nm), at a scanning speed of 2°/min from 10 to 100° (2h). Scanning electron microscope (SEM) micrographs were taken with a FEI Quanta 200 SEM operating at a 20-kV accelerating voltage. The samples for SEM were prepared by spreading a small drop containing the nanoparticles onto a silicon wafer and then drying them almost completely in the air at room temperature for 2 h, and finally transferring them onto SEM conductive tapes. Transmission electron microscopy (TEM) studies of the nanostructures were carried out with a JEOL JEM 3010 instrument operating at an accelerating voltage of 300 kV. Sonication was performed in a Shanghai Branson-BUG40-06 ultrasonic cleaner (with a frequency of 40 kHz and a nominal power of 200 W).
4.2 Preparation of the Fe3O4 nanoparticles
Fe3O4 nanoparticles were prepared using chemical co-precipitation as described in the literature [44]. Briefly, 5.83 g of FeCl3.6H2O (0.02 mol) and 2.14 g of FeCl2.4H2O (0.01 mol) were dissolved in 100 mL of deionized water at 85 °C under N2 atmosphere. Then, 10 mL of 25% NH4OH were injected into the mixture. The black magnetic nanoparticles were formed in the last step. Afterwards, the reaction mixture was cooled. The catalyst was isolated using the magnetic field and washed three times with distilled water and a solution of NaCl. The obtained nanocatalyst size was estimated around 18 nm by TEM.
4.3 General procedure for the synthesis of 5,5-disubstituted hydantoins (4a–m) by Fe3O4 nanoparticles
In a 50-mL round-bottomed flask were poured aldehyde or ketone (1 mmol), potassium cyanide (1.3 mmol), ammonium carbonate (6 mmol), and magnetic Fe3O4 nanoparticles (1 mol%) sequentially. The suspension was stirred at 70 °C in solvent-free conditions. The progress of the reaction was monitored by TLC (petroleum ether:ethyl acetate, 1:1.2 v/v). Upon completion, the mixture was cooled to room temperature and neutralized with diluted hydrochloric acid. Then, the magnetic catalyst was removed by an external magnet and reused. The obtained product was purified by crystallization from water–ethanol. The structure of the products was characterized by comparison of their physical and spectra data with those previously reported.
4.4 Spectral data of products
4.4.1 5-Phenyl imidazolidine-2,4-dione (C9H8N2O2, 4a)
White powder crystals; UV (CH3OH) λmax: 284 nm; 1H NMR (DMSO-d6, 400 MHz) δ: 10.79 (s, 1H, N3–H), 8.41(s, 1H, N1–H), 7.31 (t, J = 6.8 Hz, 1H), 7.35 (d, J = 6.89 Hz, 2H), 7.40 (t, J = 6.89 Hz, 2H) and 5.16 (s, 1H); 13C NMR (DMSO-d6, 100 MHz) δ: 173.77 (C4 = O), 157.67 (C2O), 136.49 (C), 129.09 (2CH), 128.77 (2CH), 127.11 (CH), 62.26 (Cspiro); IR (KBr cm−1)
4.4.2 5-(4-Methyl phenyl)-imidazolidine-2,4-dione (C10H10N2O2, 4b)
White crystal; UV (CH3OH) λmax: 276 nm; 1H NMR (DMSO-d6, 400 MHz) δ: 10.74 (s, 1H, N3–H), 8.35 (s, 1H, N1–H), 7.19 (s, 4H), 5.09 (s, 1H) and 2.29 (s, 3H); 13C NMR (DMSO-d6, 100 MHz) δ: 174.82 (CO), 157.97 (CO), 138.01 (C), 133.57 (2CH), 129.64 (2CH), 127.08 (C), 61.46 (C–H), 21.15 (C–H); IR (KBr cm−1)
4.4.3 5-(4-Methoxyphenyl)-imidazolidine-2,4-dione (C10H10N2O3, 4c)
White needles; UV (CH3OH) λmax: 280 nm; 1H NMR (DMSO-d6, 400 MHz) δ: 9.3 (s, 1H, N3–H), 8.34 (s, 1H, N1–H), 7.21 (d, J = 7.7 Hz, 2H), 6.94 (d, J = 7.7 Hz, 2H), 5.08 (s, 1H) and 3.74 (s, 3H); 13C NMR (DMSO-d6, 100 MHz) δ: 166.71 (CO), 160.04 (CO), 158.37 (C), 127.12 (2CH), 120.03 (2CH), 113.63 (C), 61.04 (Cspiro), 55.20 (C–H); IR (KBr cm−1)
4.4.4 5-(2-Methoxyphenyl)-imidazolidine-2,4-dione (C10H10N2O3, 4d)
White needles; UV (CH3OH) λmax: 274 nm; 1H NMR (DMSO-d6, 400 MHz) δ: 9.40 (s, 1H, N3–H), 7.99 (s, 1H, N1–H), 7.30 (d, J = 7.00 Hz, 1H), 7.08–6.92 (m, 2H), 6.85 (d, J = 7.00 Hz, 1H), 4.85 (s, 1H) and 4.01 (s, 3H); 13C NMR (DMSO-d6, 100 MHz) δ: 166.01 (CO), 161.12 (CO), 157.62 (C), 128.13 (2CH), 119.11 (2CH), 114.02 (C), 62.01 (Cspiro), 54.17 (C–H); IR (KBr cm−1)
4.4.5 5-(2-Chlorophenyl)-imidazolidine-2,4-dione (C9H7N2O2Cl, 4e)
White needles; UV (CH3OH) λmax: 275 nm; 1H NMR (DMSO-d6, 400 MHz) δ: 9.70 (s, 1H, N3–H), 8.03 (s, 1H, N1–H), 7.90 (d, J = 6.98 Hz, 1H), 7.71 (t, J = 6.98 Hz, 1H), 7.35–7.36 (m, 2H) and 5.13 (s, 1H); 13C NMR (DMSO-d6, 100 MHz) δ: 168.71 (CO), 151.04 (CO), 158.37 (C), 127.12 (2CH), 120.03 (2CH), 113.63 (C), 61.04 (Cspiro); IR (KBr cm−1)
4.4.6 5-n-Butyl-imidazolidine-2,4-dione (C7H12N2O2, 4f)
White needles; UV (CH3OH) λmax: 319 nm; 1H NMR (DMSO-d6, 400 MHz) δ: 10.52(s, 1H, N3–H), 7.89(s, 1H, N1–H), 1.49–1.52 (t, J = 5 Hz, 2H), 1.55–1.57 (m, 2H), 0.91–0.93 (m, J = 5 Hz, 2H) and 0.81–0.79 (t, J = 5 Hz, 3H); 13C NMR (DMSO-d6, 100 MHz) δ: 166.51 (CO), 158.50 (CO), 67.21 (C), 46.73 (C–H), 23.87 (C–H), 22.23 (C–H), 20.85 (C–H).; IR (KBr cm−1)
4.4.7 5-Isobutyl-imidazolidine-2,4-dione (C7H12N2O2, 4g)
White needles; UV (CH3OH) λmax: 321 nm; 1H NMR (DMSO-d6, 400 MHz) δ: 10.43 (s, 1H, N3–H), 7.74 (s, 1H, N1–H), 1.49–1.53 (m, 2H), 1.43–1.49 (m, 1H), 0.88–0.89 (d, J = 5 Hz, 3H) and 0.81–0.79 (d, J = 5 Hz, 3H); 13C NMR (DMSO-d6, 100 MHz) δ: 166.51 (CO), 158.53 (CO), 67.20 (C), 46.72 (C–H), 23.92 (C–H), 22.19 (C–H), 20.97 (C–H).; IR (KBr cm−1)
4.4.8 5-Isobutyl-5-methyl-imidazolidine-2,4-dione (C8H14N2O2, 4h)
White needles; UV (CH3OH) λmax: 312 nm; 1H NMR (DMSO-d6, 400 MHz) δ: 10.52(s, 1H, N3–H), 7.89(s, 1H, N1–H), 1.57–1.51 (m, 2H), 1.46–1.42 (m, 1H), 1.20 (s, 3H), 0.87–0.86 (d, J = 5 Hz, 3H) and 0.79–0.77 (d, J = 5 Hz, 3H); 13C NMR (DMSO-d6, 100 MHz) δ: 166.46 (CO), 158.47 (CO), 67.16 (Cspiro), 46.67 (C–H), 23.99 (C–H), 22.17 (C–H), 20.92 (C–H).; IR (KBr cm−1)
4.4.9 1,3-Diazaspiro[4,5]decane-2,4-dione (C8H12N2O2, 4i)
White needles; UV (CH3OH) λmax: 348 nm; 1H NMR (DMSO-d6, 400 MHz) δ: 10.47 (s, 1H, N3–H), 8.38 (s, 1H, N1–H), 1.62–1.45 (m, 9H) and 1.21–1.27 (m, 1H).; 13C NMR (DMSO-d6, 100 MHz) δ: 179.61 (CO), 157.51 (CO), 62.89 (Cspiro), 39.76 (2CH2), 25.33 (2CH2), 21.71 (CH2).; IR (KBr cm−1)
4.4.10 5-Methyl-5-phenyl-imidazolidine-2,4-dione (C10H10N2O2, 4j)
White needles; UV (CH3OH) λmax: 252 nm; 1H NMR (DMSO-d6, 400 MHz) δ: 10.75(s, 1H, N3–H), 8.62 (s, 1H, N1–H), 7.47–7.45 (d, J = 6 Hz, 2H), 7.40–7.36 (t, J = 6 Hz, 2H), 7.32–7.31 (t, J = 6 Hz, 1H) and 1.64 (s, 3H); 13C NMR (DMSO-d6, 100 MHz) δ: 166.16 (CO), 157.41 (CO), 127.41 (C), 127.38 (2CH), 126.79 (2CH), 123.70 (2CH), 71.22 (Cspiro), 22.14 (C–H); IR (KBr cm−1)
4.4.11 5-Methyl-5-(4-chloro phenyl)-imidazolidine-2,4-dione (C10H9ClN2O2, 4k)
White needles; UV (CH3OH) λmax: 272 nm; 1H NMR (DMSO-d6, 400 MHz) δ: 10.6 (s, 1H, N3–H), 8.64 (s, 1H, N1–H), 7.49 (d, J = 8.3, 2H), 7.47 (d, J = 8.3, 2H) and 1.63 (s, 3H); 13C NMR (DMSO-d6, 100 MHz) δ: 177.14 (CO), 156.69 (CO), 139.39 (C), 133.16 (C), 128.89 (2CH), 127.77 (2CH), 64.06 (Cspiro), 25.51 (CH3); IR (KBr cm−1)
4.4.12 5-Methyl-5-(3-methyl phenyl)-imidazolidine-2,4-dione (C11H12N2O2, 4l)
White powder; UV (CH3OH) λmax: 268 nm; 1H NMR (DMSO-d6, 400 MHz) δ: 10.73 (s, 1H, N3–H), 8.56 (s, 1H, N1–H), 7.42–7.25 (s, 3H), 7.12 (s, 1H), 2.30 (s, 3H) and 1.87–1.61 (s, 3H); 13C NMR (DMSO-d6, 100 MHz) δ: 166.16 (CO), 157.41 (CO), 137.99 (C), 131.30 (CH), 128.16 (CH), 127.31 (CH), 126.73 (CH), 123.32 (C), 72.43 (Cspiro), 22.14 (CH3), 20.84 (CH3); IR (KBr cm−1)
4.4.13 5-(3-Chlorophenyl)-5-methyl imidazolidine-2,4-dione (C10H9N2O2Cl, 4m)
White crystal; UV (CH3OH) λmax: 270 nm; 1H NMR (DMSO-d6, 400 MHz) δ: 9.86 (s, 1H, N3–H), 7.99 (s, 1H, N1–H), 7.54–7.47 (m, 2H), 7.18 (d, J = 6.5, 1H), 7.01 (t, J = 6.5, 1H) and 2.09 (s, 3H); 13C NMR (DMSO-d6, 100 MHz) δ: 176.4 (CO), 158.2 (CO), 156.3 (C), 139.0 (CH), 132.8 (CH), 128.5 (CH), 127.4 (CH), 126.2 (C), 63.7 (Cspiro), 25.1 (CH3); IR (KBr cm−1)
4.4.14 5,5-diphenylhydantoin (C15H12N2O2, 4n)
White needles; UV (CH3OH) λmax: 276 nm; 1H NMR (DMSO-d6, 400 MHz) δ: 11.1 (s, 1H, N3–H), 9.33 (s, 1H, N1–H) and 7.36 (m, 10H); 13C NMR (DMSO-d6, 100 MHz) δ: 175.26 (CO), 156.43 (CO), 140.39 (2C), 128.96 (2CH), 128.48 (4CH), 127.04 (4CH), 70.66 (Cspiro); IR (KBr cm−1)
4.4.15 Spiro[fluorene-9,4-imidazolidine]-2,4-dione (C15H10N2O2, 4o)
Yellow powder; UV (CH3OH) λmax: 250 nm; 1H NMR (DMSO-d6, 400 MHz) δ: 11.1 (s, 1H, N3–H), 9.33 (s, 1H, N1–H), 7.89–7.85 (t, J = 6.7 Hz, 2H), 7.50–7.45 (m, 4H) and 7.37–7.34 (t, J = 6.7, 2H); 13C NMR (DMSO-d6, 100 MHz) δ: 175.26 (C4O), 156.43 (C2O), 140.39 (2C), 128.96 (2C), 128.48 (4CH), 127.04 (4CH), 70.66 (Cspiro), IR (KBr cm−1)
Acknowledgements
We gratefully acknowledge the financial support from the Research Council of the University of Kashan for supporting this work by Grant No. 256722/XI.