1 Introduction
The development of controlled drug delivery systems, which led to a prolonged efficiency of the drugs, has been a focus of interest among the scientific community, which shows numerous advantages in comparison with the conventional forms of dosage. It is well established that an efficient delivery system should be able to transport the desired drug molecules with little loss and release the guest molecules in a controlled manner before reaching the targeted destinations. Different supports or methods have been used in drug delivery systems. For example, dry powder inhaler delivery (DPI) was used for poorly soluble drugs. It has one major drawback, i.e. painstaking efforts are required in the formulation steps, so that each formulation is likely applicable to only one type of drug nanoparticle [1]. Magnetic nanoparticles alone are reported to be ineffective drug carriers due to some limitations in drug loading, release rates, and retention time in the blood stream [2].
In polymer-based microsphere-controlled drug release, microspheres less than 100 μm large are suitable for intravenous delivery for absorption. When the particle size is less than 5 μm, the microspheres can be administered via inhalation. In the diffusion release method, the incorporated drug passes through the polymer pores or chains and the permeability of the polymer is dependent on environmental conditions, such as pH, temperature, ionic strength, etc. In degradation release, a polymer degrades within the human body due to a natural biological process, such as hydrolysis. Hence, the chemical structure and molecular weight are critical factors [3].
Uneven distribution of active agents in the polymer matrix is considered a limitation in optimum controlled release [4]. However, the release mechanism of the most biodegradable polymer-based drug delivery systems, based on hydrolysis, will induce an erosion of the carrier structure, and release will occur immediately after the dispersion of these systems in water, which greatly reduces drug release efficiency [5]. Also, these systems are insensitive to metabolic changes in the body and are unable to modulate drug release or target the drug to diseased tissue. This lack of control has motivated the exploitation of bioresponsive polymers as drug carriers [6].
Conventional micronization processes, such as jet milling and spray drying can result in wide size distribution, thermal denaturing, excessive surface change or roughness and hence, limiting the good control of the particle material characteristics [7–9]. In colon delivery systems, an elevation of the gastric pH is observed in elderly patients or in the patients taking H2-receptor antagonists, so that such a system may induce a dose-dumping phenomenon at undesired absorption sites. In addition, this system is not always valid, since it often suffers intra- and inter-subject variability of gastrointestinal transit time, especially gastric emptying time [10].
Despite the many advantageous properties, hydrogels have several limitations. The low tensile strength of many hydrogels limits their use in load-bearing applications and can result in the premature dissolution or flow away of the hydrogel from a targeted local site. The quantity and homogeneity of drug loading into hydrogels may be limited, particularly, in the case of hydrophobic drugs. The high water content and large pore sizes of most hydrogels often result in relatively rapid drug release, over a few hours to a few days. The ease of application can also be problematic; although some hydrogels are sufficiently deformable to be injectable, many are not, necessitating surgical implantation [11,12].
The resealed erythrocyte delivery system has disadvantages, such as: limited potential as carrier to non-phagocyte target tissue and the possibility of clumping of cells; dose-dumping may occur [13]. Liposomes are another delivery system for phagocyte-targeted therapies providing advantages, such as low immunogenicity, biocompatibility, cell specificity, and drug protection. However, there are also shortcomings, such as poor scale-up, cost, short shelf life, and, in some cases, toxicity and off target effects [14].
Several factors could affect the release profile of the hosted molecule, the nature of the host–guest chemical interaction and the pore size of the matrix among them. Regarding the influence of the pore size, the modification would be expected to be a simple way to control the delivery rate of the guest drug, owing to the well-known influence of the pore size of molecular sieves on chemical processes, involving the porous network [15]. Nevertheless, mesoporous systems present a major problem as far as drug release is concerned – limited control of drug release, which occurs mainly by diffusion [16]. The pore sizes of porous materials, such as MCM-41-type ordered mesoporous silicas, were notably larger than those of some drugs. On the other hand, for a better control of drug delivery, the pore size should be adjusted to the drug dimension. This may be achieved by decreasing the pore size by anchoring functional groups on the pore walls or synthesis materials with lower pore diameter. It has been shown that drug delivery becomes slower as the pore diameter of MCM-41-type silica decreases [17]. Nonetheless, the synthesis of ordered mesoporous materials with small pore size is difficult [7] and, therefore, the pore sizes were still too large compared to some such drugs. Besides, there are numerous drugs with smaller sizes. As an easier alternative, the zeolites have been used as suitable drug delivers, as the pore size of these microporous molecular sieves, in the range 5–7.5 Å, is closer to the size of many molecules with pharmacological activity. Taking into account the fact that in vivo and in vitro toxicity studies showed the biologically inert behavior of the natural zeolite clinoptilolite [18], zeolites appear as potential candidates for the controlled release of drugs.
To achieve an optimum storage with slow release, pore modification of the zeolite needs to occur after loading. On the other hand, for the guest molecules of pharmaceutical interest, which are usually larger than 2 nm, zeolites are not appropriate to be used as carriers due to their relatively small pore size. However, most modification methods require harsh conditions, such as high temperature, and the presence of strong acids or bases. In these cases, the encapsulated agents can be destroyed or lose their activities. Therefore, surface modification under mild condition is necessary [19]. Rivera et al. [20] have reported that the adsorption and release of sulfamethoxazol onto/from surfactant-modified clinoptilolite. In addition to the structural stability of a zeolite, a second basic chemical property is its ability to host useful pharmaceutical species, and to release them with a kinetics that suits the specific medical application. The latter is indeed a complex ability that strongly depends on the specific zeolitic material and drug under consideration. Purified natural clinoptilolite has demonstrated good stability in its transit through the gastrointestinal tract, and to be harmless to the human body [21]; the properties of the used Iranian clinoptilolite have been studied in the literature [22,23].
The presence of surfactants on solid surfaces can induce or enhance the co-adsorption of different organic molecules, due to the variation of the hydrophilic character of the specific substrate. This phenomenon is known as surface solubilization or adsolubilization, and it is ascribed to the partition of organic solutes between the aqueous phase and admicelles on the solid surface [24–26]. However, in the case of zeolites with hydrophilic characteristics (low Si/Al relation) when treated with surfactants, the composites obtained are capable of incorporating water-insoluble compounds in the hydrophobic core [27]. Considering these observations and the results reported in the literature, it is reasonable to expect the adsolubilization of drugs by surfactant/zeolite materials (SMZ), which has a great pharmaceutical attractiveness in the development of drug-support systems. In our idea, SMZs are good adsorbents for more hydrophobic drugs with respect to the hydrophilic one. In contrast, the release rate of the adsorbed drug is faster in the adsorbed hydrophilic system with respect to the hydrophobic one.
Cephalexin, or 7-[(aminophenylacetyl)amino]-3- methyl-8-oxo-5-thia-1-azabicyclo [4.2.0] oct-2-ene- 2-carboxylic acid as a semi-synthetic drug is the most widely used cephalosporin antibiotic [28,29].
In this work, an Iranian clinoptilolite tuff was pretreated (removing of obvious solid impurities) and crushed by a mechanical method to obtain micro sized particles and then, changed into nanoparticles during a ball milling process. The obtained nanoparticles were modified with HDTMA to obtain surfactant-modified zeolite (SMZ). The resulting SMZ was used for the adsorption of cephalexin in an aqueous solution and the effects of some key operating parameters, such as: loaded extent of HDTMA onto zeolite surface, solution pH, dosage of SMZ, contact time, etc., were studied on the adsorption extent of cephalexin. Hence, optimized conditions were obtained to reach the adsorption extent of cephalexin onto SMZ. Finally, some SMZ–CPX systems were prepared in optimized conditions and used in delivery experiments. The effects of some experimental factors, such as delivery time, solution pH and the presence of inorganic cations (such as: Fe2+, Fe3+, Na+, K+, Ca2+, Mg2+), on the delivery extent of cephalexin from the SMZ–CPX system were studied.
2 Experimental
2.1 Materials
The natural zeolite was obtained from Semnan Province in the northeast of Iran. Hexadecyltrimethylammonium bromide (HDTMA-Br) was purchased from Aldrich chemicals (Milwaukee, WI), and was used without further purification. Cephalexin (CPX) and other used salts were purchased from Merck and used as received. Distilled water was used throughout the experiments. The pH of the solutions was appropriately adjusted with an NaOH or HCl solution (in the case of unbuffered solutions) and NaOH, HCl and phosphoric acid solutions and phosphate salts (in the case of buffered solutions).
2.2 Pretreatment of the raw zeolite and the preparation of nano-clinoptilolite powder
Natural clinoptilolite tuffs were mechanically pretreated, by crushing in an agate mortar and sieving in analytical sieves, for separating the particles ≤ 100 μm. The obtained powder was used to prepare the nanoparticles of zeolite using a planetary ball mill (PM100; Retsch Corporation). To optimize milling conditions with respect to size reduction and crystallinity retention, milling parameters, such as rotational speed, ball-to-powder ratio as well as the grinding time were varied for different experiments. In most of these tests, powders with particle sizes smaller than 100 μm were used in dry milling during 6 h. All of the tests were done in a 250-mL stainless steel jar with a protective jacket of polymer. Zirconium oxide balls of 20 and 3 mm were used for dry and wet millings, respectively. The grinding jars were arranged eccentrically on the sun wheel of the planetary ball mill. The direction of movement of the sun wheel being opposite to that of the grinding jars was selected with the ratio of 1:1. Due to lack of appropriate accessories to control the temperature and pressure of the jar during grinding, sampling was carried out at the end of this period, at which time the jar was allowed to cool down to room temperature [30]. In order to remove any water-soluble impurity – and also the magnetic ones, – the obtained nanoparticles were heated at 70 °C in distilled water under magnetic stirring for 24 h, while renewing daily the water content. In order to reach a fixed water content, after filtration, washing and drying, the pretreated powder was stored in a desiccator over a saturated sodium chloride solution for 2 weeks.
2.3 Preparation of the SMZ and SMZ–drug
To prepare the SMZ, 2 g of clinoptilolite nanoparticles were added to 50-mL aqueous solutions of the surfactant at different concentrations (50, 100, and 200 mM) in polyethylene bottles. The samples were shaken with a reciprocating shaker for 24 h. The suspensions were centrifuged at 3000 rpm for 15 min. Surface-modified samples were then filtered and washed with excess amounts of water until no foam was formed by shaking the supernatant. The prepared SMZ was air-dried for 72 h.
The liquid phase adsorption of the model guest compounds (CPX) was carried out at 15 different concentrations of the drug (0.004–1.28 mM). Different weights of SMZ were treated with 25 mL of the drug solutions at room temperature and agitated at a wide range of time and pH values.
2.4 Structural characterization and instruments
To study the probable changes of morphology for ground powders after ball milling, the samples were subjected to SEM investigation (Seron technology, model: AIS2100). Nano-clinoptilolite samples were analyzed by X-ray diffractometry (Bruker, Diffraktometer D8 Advance, Germany) using Cu Kα as the radiation source and Ni as the filter. The crystallite size of the particles was calculated from the line broadening of the main diffraction lines using the Scherrer equation as follows:
d = kλ/β1/2 cosθ | (1) |
TG and DTG were recorded for the samples using Model Setaram (STA) units in the 50–600 °C range, with a temperature rise of 10 °C min−1. The sensitivity of the thermobalance was ± 1 μg. About 35 mg of each sample were used in the test. Fourier transform–infrared spectroscopy was carried out by using a Nicolet 400D Impact at wavenumbers from 400 to 4000 cm−1 by using KBr pellet (here only the 2000–400 cm−1 range was shown). The microstructure of nano-clinoptilolite particles was recorded using transmission electron microscope S-3500 N with absorbed electron detector S-6542 (Hitachi Science System Ltd).
For the studies of adsorption in the liquid phase, the drug concentrations in solution before and after the contact with the SMZ (or SMZ–CPX in delivery experiments) were followed by ultraviolet spectroscopy (UV) according to the procedure reported in the literature [32]. The UV spectra were collected by means of a Carry 100 spectrophotometer in the wavelength interval 200–400 nm.
2.5 Drug desorption
Drug desorption from the SMZ–CPX system was studied by soaking 0.1 g of SMZ–CPX in water in a wide range of time and pH values under constant stirring at 150–200 rpm. Drug release was also studied in the presence of some other ions (Fe2+, Fe3+, Na+, K+, Ca2+, Mg2+). The drug concentrations in solution as a function of the stirring time were measured with a UV–Vis spectrophotometer.
3 Results and discussion
3.1 Chemical and physical characterization of the samples
3.1.1 Surface morphology and TEM studies
Some of the SEM images of the NZ and SMZ samples are shown in Fig. 1. SEM images indicated that almost in all the samples, zeolite powder with particles size less than 100 nm could be recognized as a separated particle or in the form of larger agglomerates. Moreover, most particles have lost their initial layered shape and have been converted into spherical, elliptical or irregular shapes. By careful considerations of SEM images, some crystals with sharp edges and clean surfaces were observed; they are about 100–200 nm in size. Therefore, it can be concluded that careful selection of milling conditions may result in the production of nano-clinoptilolite zeolite with the desirable crystalline structure. Nevertheless, mechanical production of zeolitic nanoparticles by means of planetary ball mills may also reduce zeolite crystallinity. To have more precise information about the particle size of the prepared sample, TEM photographs of nanoparticles of clinoptilolite were also taken (Fig. 2h). As shown, the major particles of clinoptilolite particles have sizes less than 100 nm.
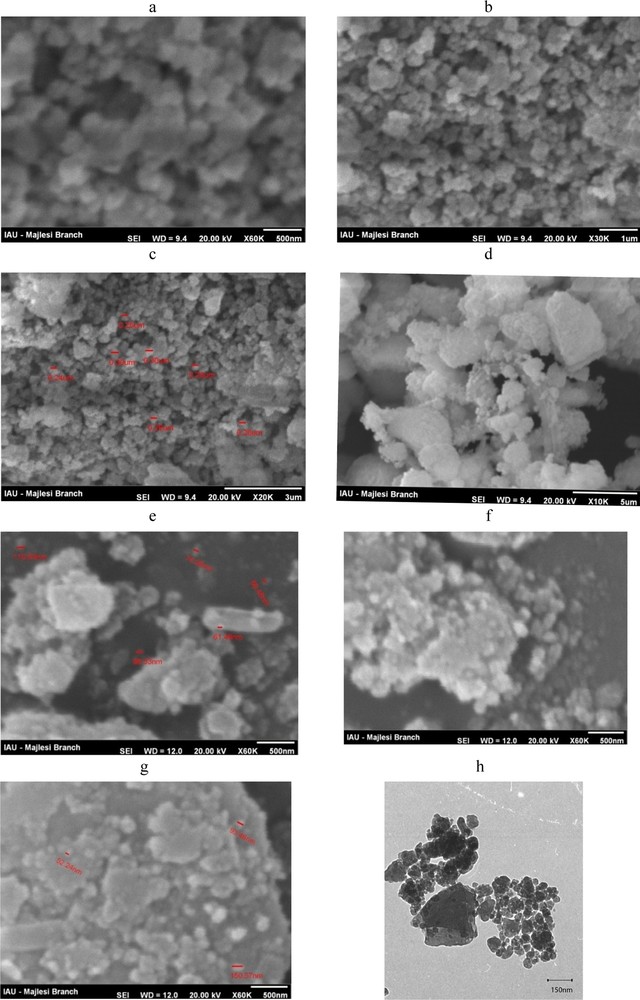
Scanning electron microscopy (SEM) images of NZ (a–c) and SMZ (d–g) and TEM image of NZ (h).
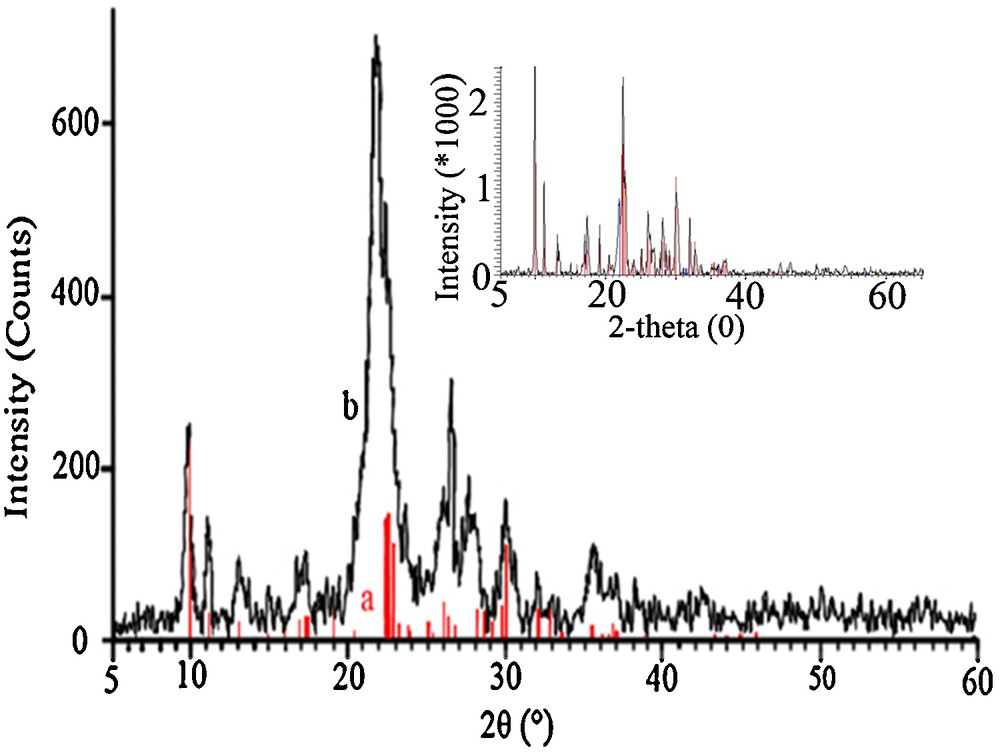
X-ray diffraction (XRD) spectrum of nano-clinoptilolite and clinoptilolite (inset).
3.1.2 XRD investigation
The XRD patterns of nano zeolite (NZ) and also clinoptilolite in micro dimensions (inset of Fig. 2) were obtained and displayed in Fig. 2. Clinoptilolite was characterized with the strong diffraction peaks located at 2θ degree values of 9.8 and 22.3, corresponding to the d020 and d004 reflections [33,34]. The observed reflection peaks can be indexed to clinoptilolite crystalline structure data in the instrument's library [the lines in the XRD patterns of Fig. 2 with JCPDS No. 39-1383]. Good agreement between the results shows that the original natural zeolite includes clinoptilolite structure as its major component. XRD patterns also showed that the used zeolite tuffs include slight amounts of quartz (3.5%) and cristobalite (7.8%) phases as impurities. Comparing the XRD patterns of nano-clinoptilolite particles with that of micro dimension one (inset of Fig. 2) confirms that the crystallinity and hence, the intensity of the nano-sample decreased with respect to the original parent clinoptilolite. Our results are in agreement with a ball milling investigation of natural clinoptilolite in the literature [30], which showed that the loss in crystallinity depends on the ball milling conditions, including dry and wet milling durations, rotational speed, balls-to-powder ratio and water-to-powder ratio.
The presence of broad lines in the XRD pattern of the prepared nano-clinoptilolite particles agrees with the formation of nanoparticles during the ball milling process. So, the average particles size of the samples was determined using the Scherrer equation [31]. With a small particle size of less than 100 nm, significantly wider peaks in the XRD spectrum were observed. Using the Scherrer equation, the average size of the used clinoptilolite particles was obtained in the 65–78 nm range.
Some authors compared the particles sizes of synthesized zeolites by XRD and SEM. For example, Castagnola et al. obtained particle sizes of 250 and 13 nm by SEM and XRD methods, respectively, for nanometer-sized zeolite X. They concluded that the nanoparticles of zeolite X tend to aggregate, which increases the particle sizes observed by SEM. So, they reported, directly controlling the diffraction peak's broadening by the size, that XRD provides a better estimate of the true size of these crystallites [35]. The ZSM-5 particles ZSM-5 (15 nm by XRD), ZSM-5 (60 nm by XRD) and zeolyst ZSM-5 grow into larger aggregates, as shown by the SEM images, and it is therefore difficult to determine the particle size from the SEM images. The larger aggregates ranges from 100–200 nm for ZSM-5 (15 nm) to 400–600 nm for ZSM-5 (60 nm) to 700–1000 nm for zeolyst ZSM-5 were obtained by SEM [36]. It should be mentioned that abundant intergrowth was observed in most of the samples, which makes it difficult to evaluate the dominant population of nanoparticles by SEM [37].
3.1.3 Thermal investigation of the samples
It is a known fact the surfactants can be adsorbed from aqueous solutions on surfaces with an opposite charge to that of the surfactant [38,39]. As mentioned above, clinoptilolite shows a strong preference for the cationic surfactant, such as HDTMA. We expected that the incorporation of HDTMA onto clinoptilolite is carried out through ion exchange and hydrophobic interactions, as it has been established from the adsorption of cationic surfactants on surfaces with negative charge density [40,41]. The occurrence of HDTMA on the zeolite surface is the result of the external diffusion stage of an ion exchange process, which is in agreement with the cationic nature of the surfactant [42,43], and the impossibility of these molecules to enter the channels of the clinoptilolite due to their dimensions. Hence, the loading extent of HDTMA onto the NZ and also CPX onto the obtained SMZ was determined by the gravimetric method. The TG/DTG curves for NZ, SMZ and HDTMA were obtained; the results are depicted in Fig. 3. In the case of NZ, a continuous weight loss in the studied temperature range was observed. The weight loss between 50 and 200 °C is associated with the physically adsorbed water. According to the literature, at higher temperatures, water coordinated to the zeolite cations is steadily lost [44]. In TG diagrams of the SMZ, an initial weight loss until 200 °C associated with water desorption and a weight loss between 200 and 300 °C corresponding to the surfactant decomposition were observed. This is confirmed by the results of the DTG curves, where mass loss peaks appear around 242 °C, in agreement with the peak that appears in the DTG curve for the surfactant alone. Therefore, the difference in mass loss between NZ and the SMZ, in this region, can be related directly to the surface content of surfactant. These results are similar to those obtained by Gener-Batista [45] and Vujaković et al. [46].
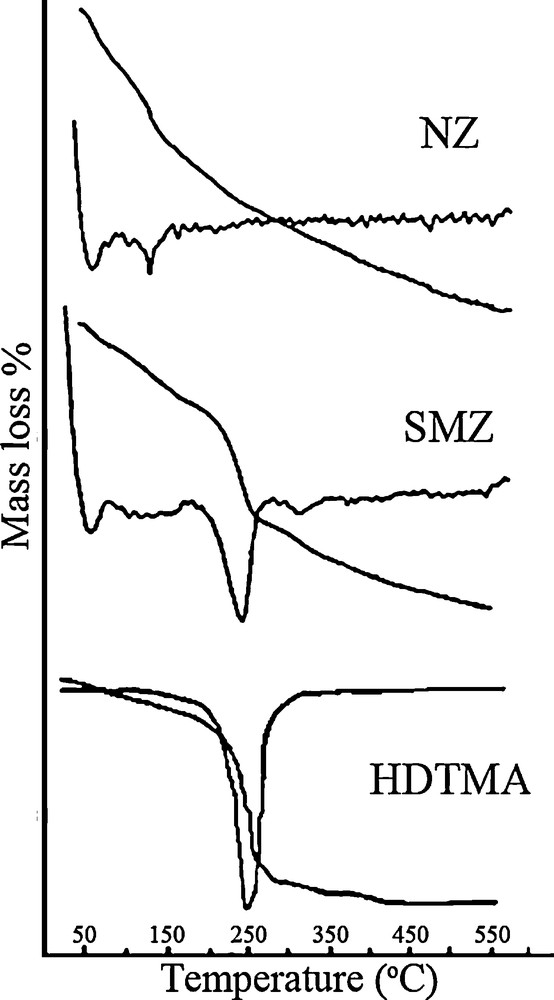
TG and differential thermogravimetry (DTG) curves for NZ, SMZ, and HDTM.
Fig. 4 shows TG/DTG curves for cephalexin (CPX), SMZ and SMZ–CPX. The cephalexin displays three weight loss peaks at 72, 195 and 303 °C. The first one corresponds to the oxidation of the compound, while the two other ones correspond to the final thermal decomposition, which occurs in two steps [47]. According to the literature [48], cephalexin relatively shows the same thermal behavior in both air and nitrogen atmospheres, so it shows a clear decomposition step in a very narrow range. It shows an exothermic peak in DTA analysis, even in nitrogen, due to the presence of enough oxygen atoms in the molecule for the beginning of an intra molecular oxidation [48]. The SMZ–CPX system shows three peaks at 60, 230 and 307 °C. The peak at 307 °C can be assigned to the last step of the decomposition process of cephalexin, which evidences its presence in the composite. The mass losses that take place at 60 and 230 °C are due to HDTMA and cephalexin decomposition, respectively. However, two types of shifts appear in these peaks. In the first place, by comparing the DTG curves of the SMZ with those of SMZ–CPX system, it is observed that an increase takes place in the temperature of the decomposition peak of the surfactant after the interaction of the material with cephalexin. In the second place, comparing the DTG curves of cephalexin and those of the SMZ–CPX system confirms a shift of the peaks corresponding to the drug towards lower temperatures, which occurs after the surfactant has been decomposed. The weight loss extents of samples are summarized in Table 1. Finally, TG/DTG results show loading sufficient amounts of HDTMA and cephalexin onto nano-clinoptilolite and the resulting SMZ, respectively.
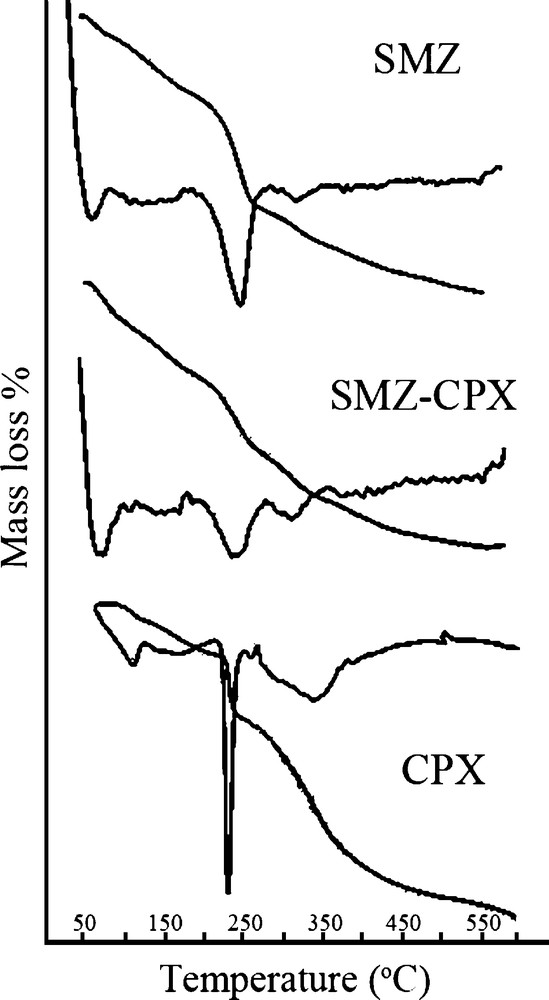
TG and differential thermogravimetry (DTG) curves for SMZ, SMZ–CPX, and CPX.
Mass loss of our samples analyzed by TG and differential thermogravimetry.
Samples | Mass loss (%) |
NZ | 9.2 |
HDTMA | 31.5 |
SMZ | 12.6 |
SMZ–CPX | 14.4 |
CPX | 71.1 |
3.1.4 FT–IR investigation
The FT–IR transmittance spectra of NZ, SMZ, HDTMA, CPX and SMZ–CPX are shown in Fig. 5. The vibration modes of the zeolitic samples are assigned by Flanigen et al. [49] and Alonso [50]. No relevant variations in the wavenumbers of the bands assigned to the zeolite after the treatment with HDTMA were observed. This indicates that the zeolite structure remains unaltered after the modification. However, three new bands appear, which correspond to the HDTMA present on SMZ: two bands associated with the C–H stretching vibrations of the hydrocarbon chain (2922 and 2652 cm−1), and a third band corresponds to the C–H bending of the methyl and methylene groups (1486 cm−1) [51]. The vibration modes of the adsorbed HDTMA on NZ do not show significant shifts compared to the spectrum of HDTMA, indicating weak interactions between the hydrocarbon chain of the surfactant and the zeolite surface [52]. The major IR peaks observed in cephalexin were located at wavenumbers of 3485 (3300–3500) (N–H), 1760 (1680–1760) (CO), 3056 (2500–3300) (O–H), 2593 (2590–2550) (S–H), 1196 (1220–1020) (C–N), and 1282 (1000–1300) cm−1 (C–O) [53]. Finally, it is important to note that the characteristic bands of the cephalexin are interfered with the main band of the matrix, thus, it was not possible to detect the presence of the drug on the SMZ–CPX systems. The small amount of cephalexin incorporated on the SMZ makes its detection difficult. In addition, some important bands of the cephalexin molecule appear at the same vibration frequencies as the SMZ bands. However, the use of other characterization techniques allowed its detection, as it was shown by TG/DTG results. These results are similar to those reported in the literature for sulfamethoxazole [20] and metronidazole [54].
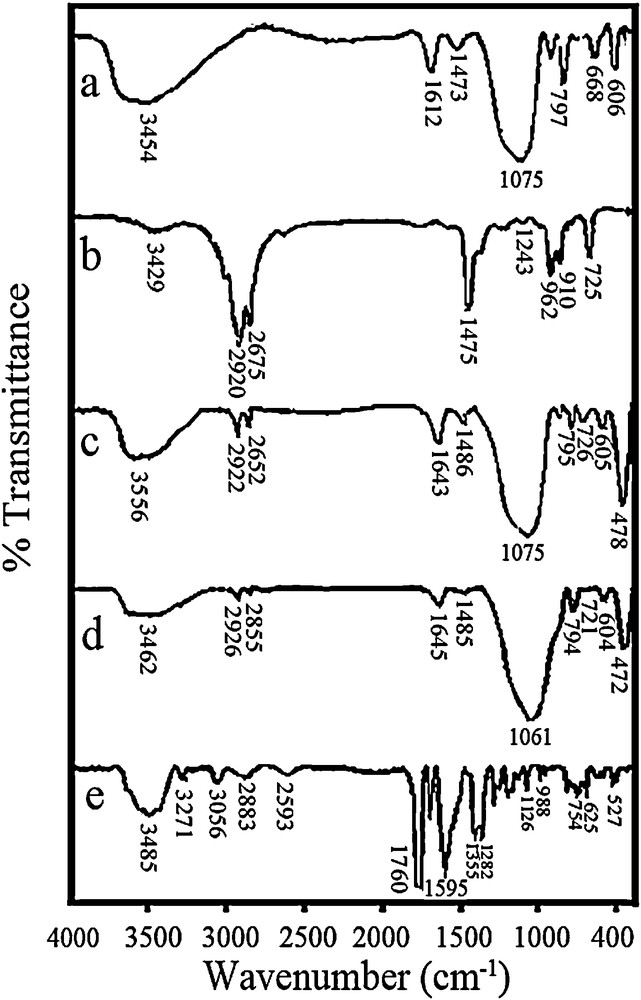
IR transmittance spectra for NZ (a), HDTMA (b), SMZ (c), SMZ–CPX (d) and CPX (e).
3.2 Drug adsorption studies
As shown in the literature, the amount of adsorbed drug by the raw or unmodified zeolitic materials is negligible [50,54]. It is also demonstrated that the polarity of the adsorbed molecules and the nature of the solid surface play an important role in the adsorption efficiency. Based on these points, we changed a normal-sized clinoptilolite to a nano-sized sample to increase the effective surface area of the solid surface. This, in turn, increases the loading extent of the cationic surfactant molecules onto the surface of the zeolite as the result increases the adsorbed drug extent. Here, the effect of the experimental parameters on the adsorption extent of cephalexin onto the proposed SMZ will be discussed.
3.2.1 Effect of surfactant on the adsorption extent of the drug
As we know, zeolites are good mineral cation exchangers and due to their definite pores size, they act as shape- and size-selective exchangers. Hence, in an ion exchange process, including cationic surfactants, these large cations only occupy the surface ion exchange sites of zeolites [55,56]. A mono- and bilayer of surfactants can be formed onto the zeolite surface at lower and higher concentration than its critical micelle concentration (CMC). In the latter case, a surfactant-modified zeolite (SMZ) is produced, with a charge reversal of the zeolite from negative into positive. The obtained SMZ acts as an anion exchanger, which adsorbs also some organic molecules between the organic chains of the double layer. The concentration of the surfactant solution plays a major role in the adsorption or anion exchange capacity of the related SMZ [55,56]. To study the effect of the modification of the zeolite surface and also the role of the surfactant concentration on the adsorption extent of the used nano-clinoptilolite, some experiments were performed by raw NZ and surfactant-modified nano-clinoptilolite, which were prepared with 50, 10 and 200 mM of HDTMA surfactant, and thereafter referred to as SMZ-1, SMZ-2 and SMZ-3, respectively.
As shown in Fig. 6, the ability of SMZ to adsorb cephalexin is more than that of raw natural zeolite. The results also show that SMZ-1 has the best adsorption capacity. It seems that in this concentration, the surfactant admicelle are completed on the surface of zeolite and the ability of SMZ to adsorb the drug will be increased. Our results are in accordance with the literature. For example, similar results were observed in our previous works in potentiometric determination of some anions by modified membrane and also carbon paste electrodes with different SMZs [57–59]. It has also been found when the HDTMA concentration was more than twice the concentration of the external CEC (or its ECEC), the zeolite absorbed 84% of the initial HDTMA concentration, while it adsorbed about 94% of the initial concentration when the HDTMA concentration was two-fold that of the ECEC [60]. In addition, the same SMZs were prepared by natural micro sized clinoptilolite and the results showed that they have a 10–20% decrease in adsorption capacity with respect to related nano scale SMZs. This is linked to an increase in the effective surface area of the nano-sized sample. According to the results, SMZ-1 was used as the best adsorbent in the next studies.
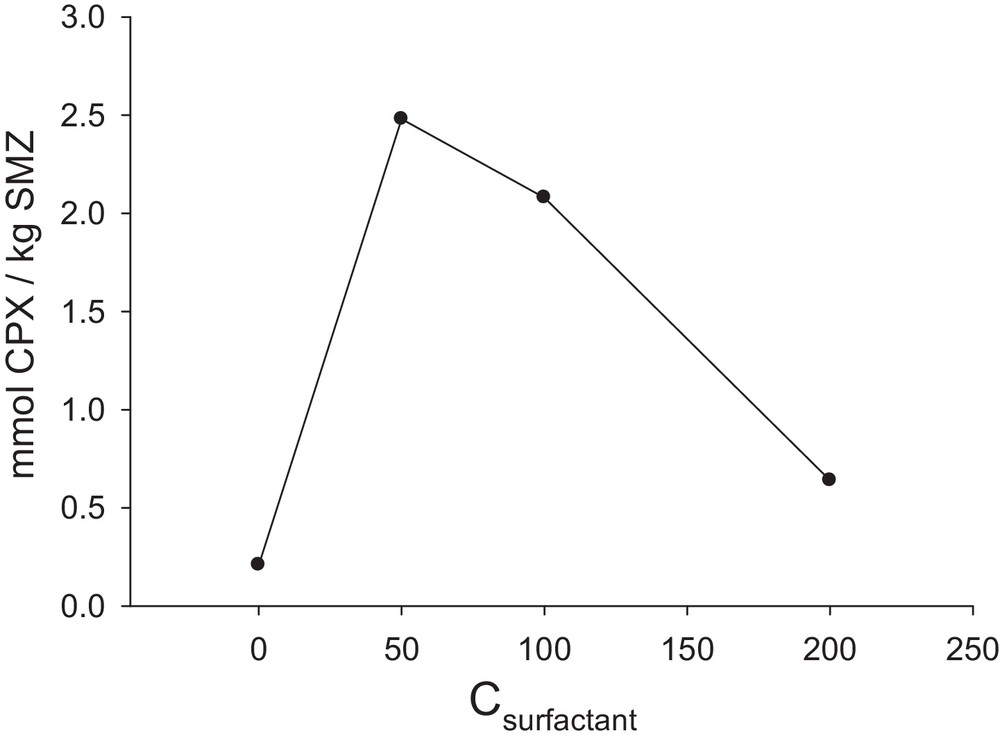
Amount of cephalexin adsorbed per unit mass of SMZ on NZ in different concentration of surfactant adsorbed on NZ (SMZ-1 = 50 mM HDTMA, SMZ-2 = 100 mM and SMZ-3 = 200 mM) and pure NZ; CCPX = 0.08 mM; time = 3 h; 4 g nanozeolite L−1; pH = 9.
3.2.2 Effect of contact time on the adsorption extent of the drug
Due to the equilibrium nature of the adsorption process, the contact time is important to reach the process at the equilibrium state. Hence, the modified nano-clinoptilolite was put in contact with the solution of 0.08 mM cephalexin (pH = 9) at different times; the results are collected in Fig. 7. The results of two repeated experiments showed that by increasing the contact time from 3 to 6 h, the adsorbed drug extent increased. In this time interval, the active sites of the used SMZ were occupied gradually and finally saturated at 6 h. It is possible that some adsorbed drug molecules tend to desorb and hence, at longer contact times than 6 h, the adsorbed drug extent decreases. In the next experiments, a contact time of 6 h was used.
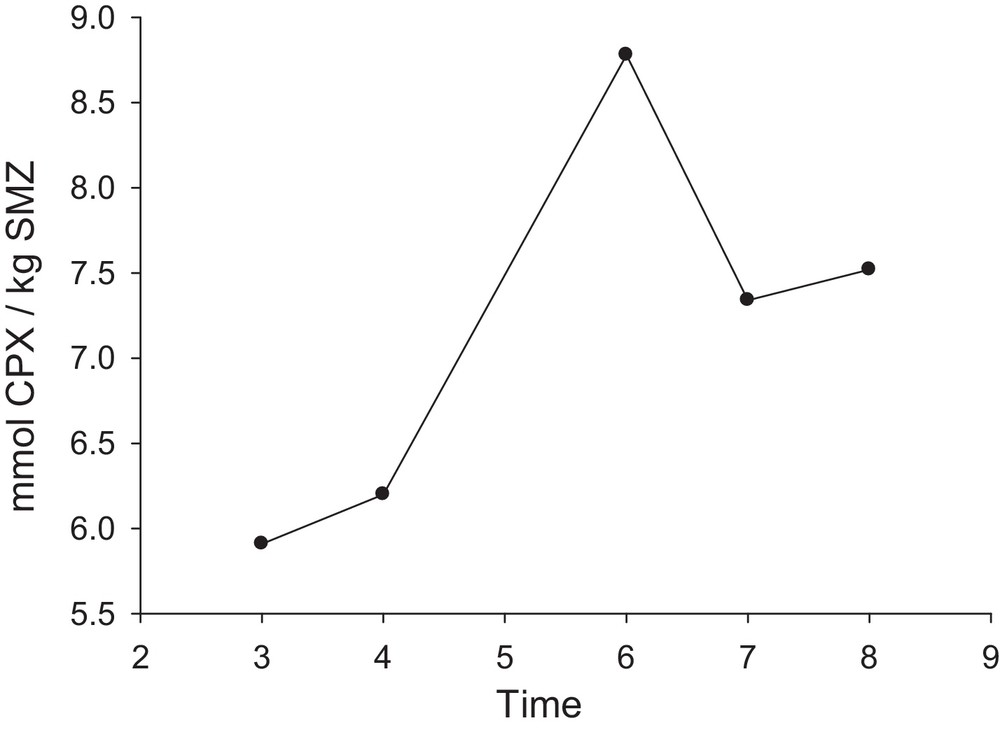
Amount of cephalexin adsorbed per unit mass of SMZ as a function of time (t1 = 3 h, t2 = 4 h, t3 = 6 h, t4 = 7 h, t5 = 8 h); SMZ = SMZ-1; 25 mL of CPX solution; CCPX = 0.08 mM; 4 g SMZ-1 L−1; pH = 9.
3.2.3 Effect of the amount of zeolite on the adsorption extent of the drug
Adsorbent dose plays an important role in the adsorption and also in ion exchange processes. An increase in the adsorbent amount brings more active sites into contact with the medium and hence, more drug can be adsorbed at the surface of the adsorbent. Against, at higher adsorbent doses, some adsorbent particles tend to aggregate, which results in a decrease of the number of active or available surface sites and hence, a decrease in the adsorbed drug extent. To study these effects, some experiments were performed in suspensions containing 0.005 to 0.2 g of SMZ in 25 mL of a 0.08 mM CPX solution at pH = 9 for a contact time of 6 h. The results that are depicted in Fig. 8 are in accordance with our speculation, and show the best results when 0.1 g SMZ was used.
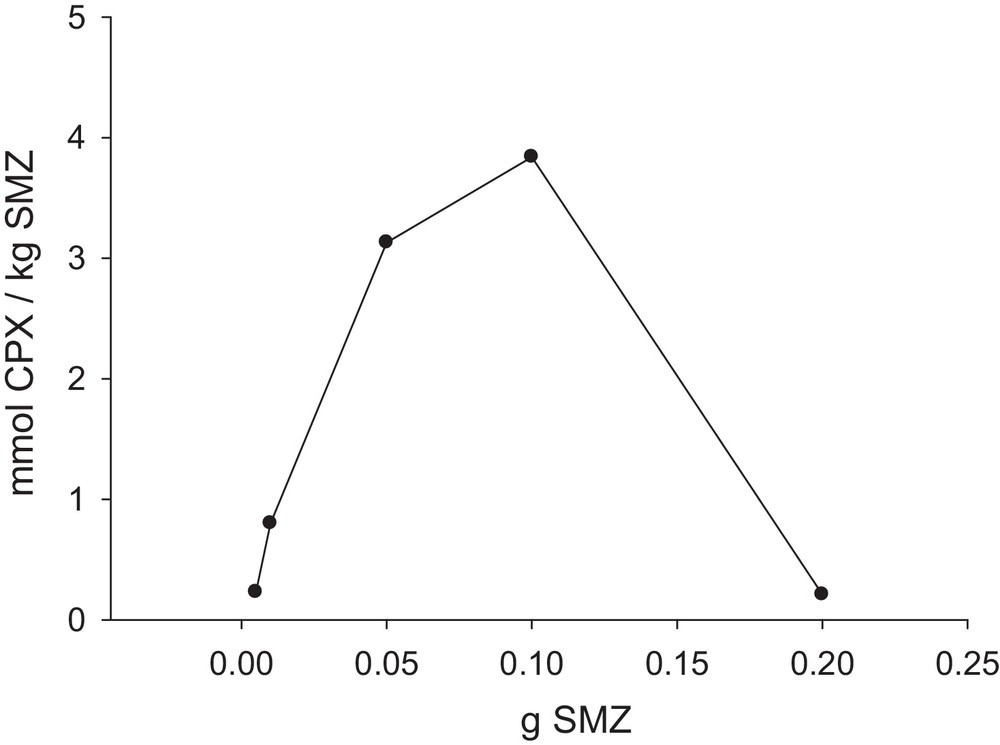
Amount of cephalexin adsorbed per unit mass of SMZ as a function of the quantity of SMZ (m1 = 0.005 g, m2 = 0.01 g, m3 = 0.05 g, m4 = 0.1 g, m5 = 0.2 g); SMZ = SMZ-1; time = 6 h; 25 mL of CPX solution; CCPX = 0.08 mM; pH = 9.
3.2.4 Effect of the concentration of cephalexin on the adsorption extent of the drug
The concentration of the analyte affects its adsorption extent due to the equilibrium nature of the adsorption process. The effect of different concentrations of cephalexin was studied in suspensions containing 0.1 g of SMZ in 25 mL of CPX solutions at pH = 9 for a contact time of 6 h. The obtained results (Fig. 9) show small adsorption extents at very low or very high concentrations. But in the range from 0.004 mM to 0.32 mM, a steep increase of the drug adsorption was observed and thereafter decreased. Hence, 0.32 mM of cephalexin was selected for the next studies. With increasing the concentration of cephalexin, the amount of adsorbed drug will be increased until the certain concentration necessary for all the available pores is filled and the surface of the zeolite is saturated. After a certain concentration (0.32 mM), a decrease in the adsorption extent was observed. We suggest that, at concentrations higher than 0.32 mM, hydrogen bonding occurs between the carbonyl and amine groups of the adjacent cephalexin molecules, which causes the formation of semi dimmer molecules with larger size than that of the single one. So, these larger molecules do not enter between the double alkyl chains of sorbed HDTMA onto the zeolite surface and drug adsorption decreases.
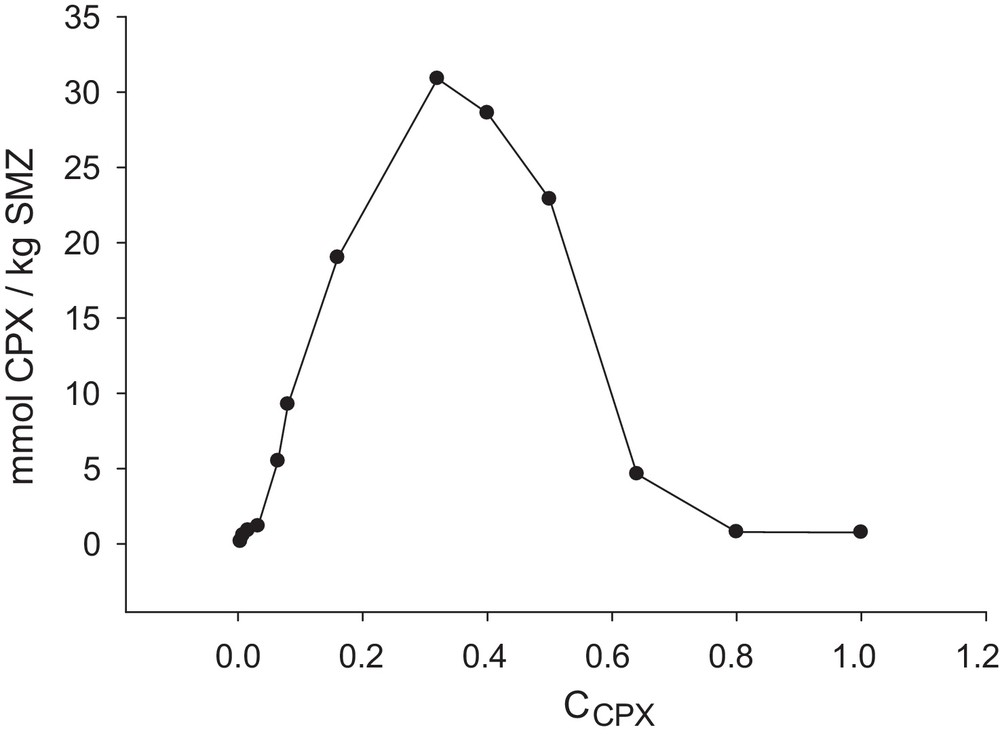
Amount of cephalexin adsorbed per unit mass of SMZ as a function of the concentration of drug (CPX1 = 0.004 mM, CPX2 = 0.008 mM, CPX3 = 0.016 mM, CPX4 = 0.032 mM, CPX5 = 0.064 mM, CPX6 = 0.08 mM, CPX7 = 0.16 mM, CPX8 = 0.32 mM, CPX9 = 0.40 mM, CPX10 = 0.50 mM, CPX11 = 0.64 mM, CPX12 = 0.80 mM, CPX13 = 1.00 mM); SMZ = SMZ-1; time = 6 h; m = 0.1 g; pH = 9.
3.2.5 Effect of the pH of the solution on the adsorption extent of the drug
It is desirable that the pH of the cephalexin solution plays the most important role in its adsorption onto the used SMZ due to the presence of both carboxylic acid and amine groups as the acidic and basic functional groups in its structure, respectively. Hence, the effect of the pH of the cephalexin solution, covering the range from 2 to 12, on the efficiency of the process was investigated and the obtained results are summarized in Fig. 10. As our results show, when the pH of the cephalexin solution decreases, the amount of the drug adsorbed by SMZ diminishes. At strong acidic pHs, the protonation of the amine groups decreases the attractive forces between the non-bonding electrons of the amine groups and the positive charges on the ammonium head group of the surfactant on the double layer of SMZ. Hence, by increasing the pH, this repulsive force decreases and the adsorbed drug extent increases. In addition, in these conditions, chloride anions compete with cephalexin species to occupy the positive sites onto the SMZ. As the results show, the most adsorbed cephalexin onto SMZ can be seen at pH = 12. At pH 12, due to the dissociation of the carboxylic acid functional group of cephalexin, the formation of anionic groups on cephalexin increases the attractive forces between these anionic groups and the positive charges of the bilayer on SMZ, which causes a sharp increase in cephalexin adsorption on the positive surface of the modified zeolite.
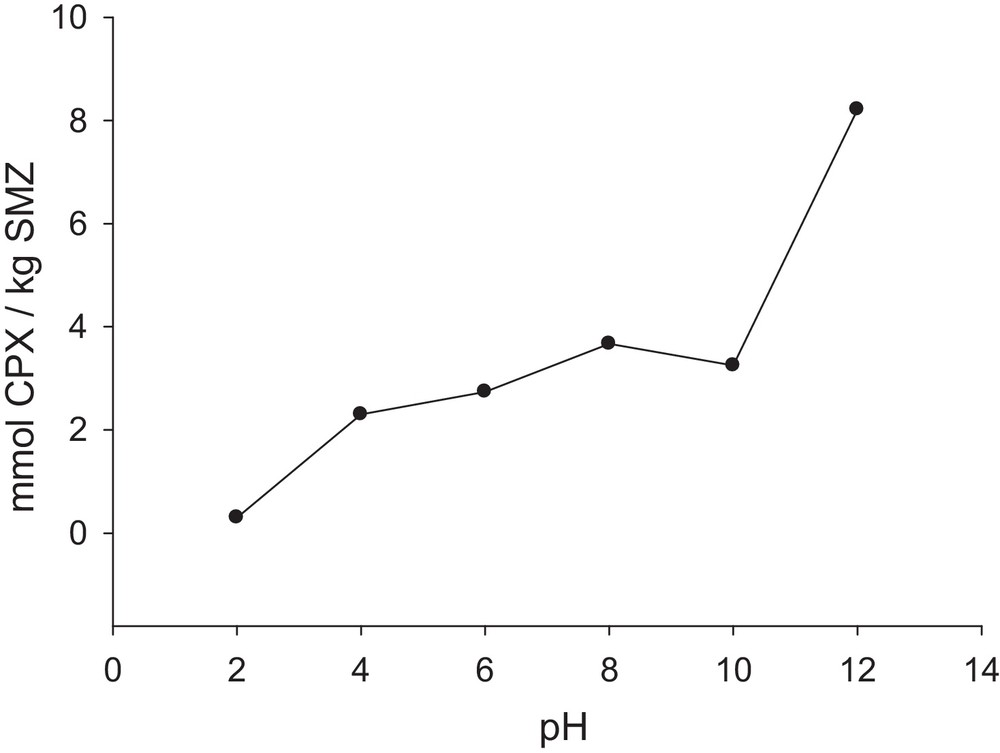
Amount of cephalexin adsorbed per unit mass of SMZ as a function of pH values (pH1 = 2, pH2 = 4, pH3 = 6, pH4 = 8, pH5 = 10, pH5 = 12); SMZ = SMZ-1; time = 6 h; m = 0.1 g;. CCPX = 0.32 mM.
The toxicity of HDTMA has been studied in the literature [61]. In our studies, the leached HDTMA from the SMZ is negligible because of its low solubility in water, and it is less than its LD50 value (410 mg/kg). To confirm this, the released HDTMA from 0.1 g SMZ–CPX and SMZ systems in 10 mL water (pH = 2) after 16 h was determined by comparing the conductance of the cleaned solution with 0.01 mM of HDTMA and a blank (10 mL of water at pH = 2) solution in the same conditions. The results showed that the conductance of the released solution changed only by 2 μΩ with respect to the blank solution, which confirms the low leaching of HDTMA in the used conditions.
3.3 Drug desorption studies STOP le 9/08/13
3.3.1 Effect of the pH of the solution on the desorption extent of cephalexin
The effect of the solution's pH on cephalexin desorption is shown in Fig. 11, which shows that when the pH of the used suspension decreases, the amount of the desorbed drug from SMZ increases. This increase in the released drug is due to the presence of a Cl– ion produced by the added hydrochloric acid, which can replace the drug onto the SMZ, whereas in the alkaline conditions, the anionic COO– group of cephalexin is formed, which prefers to adsorb onto the SMZ and hence, decreases the drug desorption. As the results show, the acidic pH of the stomach is appropriate for drug delivery. Desorption of cephalexin was also studied in different phosphate buffer solutions. As shown in Fig. 11, with increasing the pH, the released CPX was increased due to increasing the triple-, di- and mono-valent phosphate species in the solution. On the other hand, with increasing the charge of the formed phosphate species by increasing the pH, the tendency of the produced anionic species to occupy the active sites onto the SMZ will increase and hence, the desorbed amount of CPX was increased.
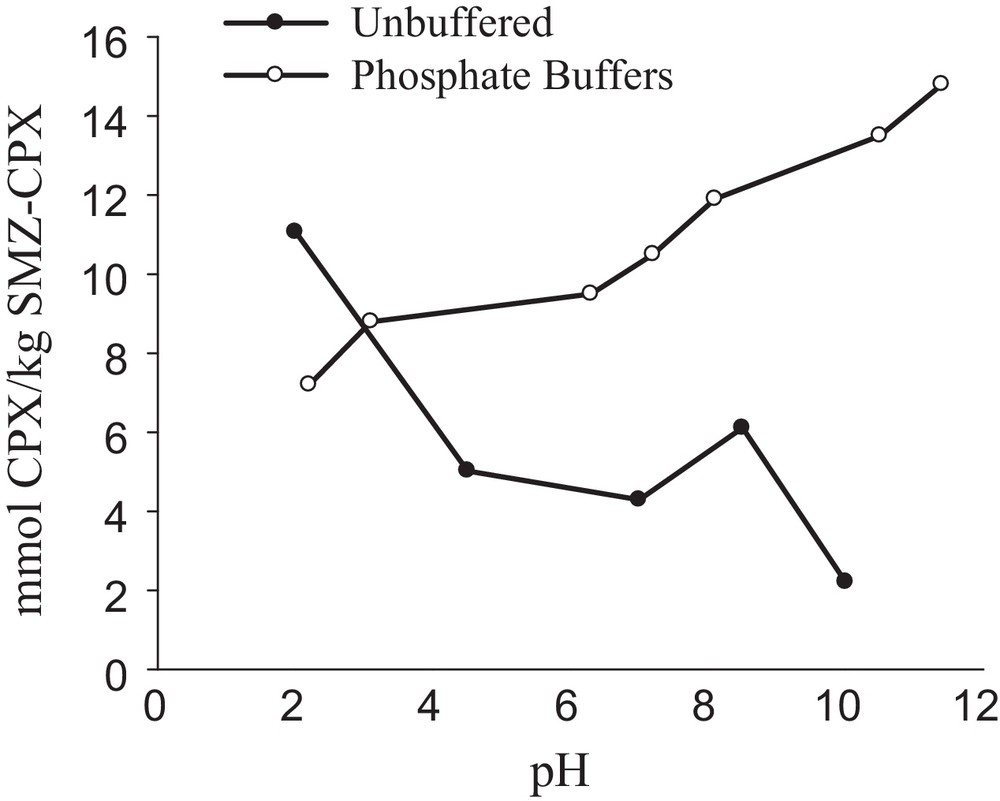
Amount of cephalexin released from unit mass of SMZ–CPX as a function of pH (in both buffered and unbuffered solutions); 2 g SMZ–CPX L−1; time = 13 h.
3.3.2 Effect of time on the desorption extent of the drug
The release of the drug on a delivery process can be treated as follows: before releasing the drug, the released fluid must penetrate into the pores or between the alkyl chains of the bilayer of the covered surfactant present on the SMZ surface, and then the adsorbed drug must dissolve into the permeating fluid, and finally, diffuse from the system [62]. Fig. 12 shows the time-dependent release rate of cephalexin from the SMZ–CPX system at pH = 2. The slow release of cephalexin, which occurs in long periods, can be linked to the co-release of the drug. This is due to its polarity with the area where two hydrophobic surfactant layers have gathered. Hence, it causes the adsorption of drug within the two micelle layers, releasing the drug in a longer time, and to drug diffusion from the core of the surfactant admicelle. The rapid liberation could be attributed to the diffusion of the drug mainly partitioned on the edges of the surfactant aggregates.
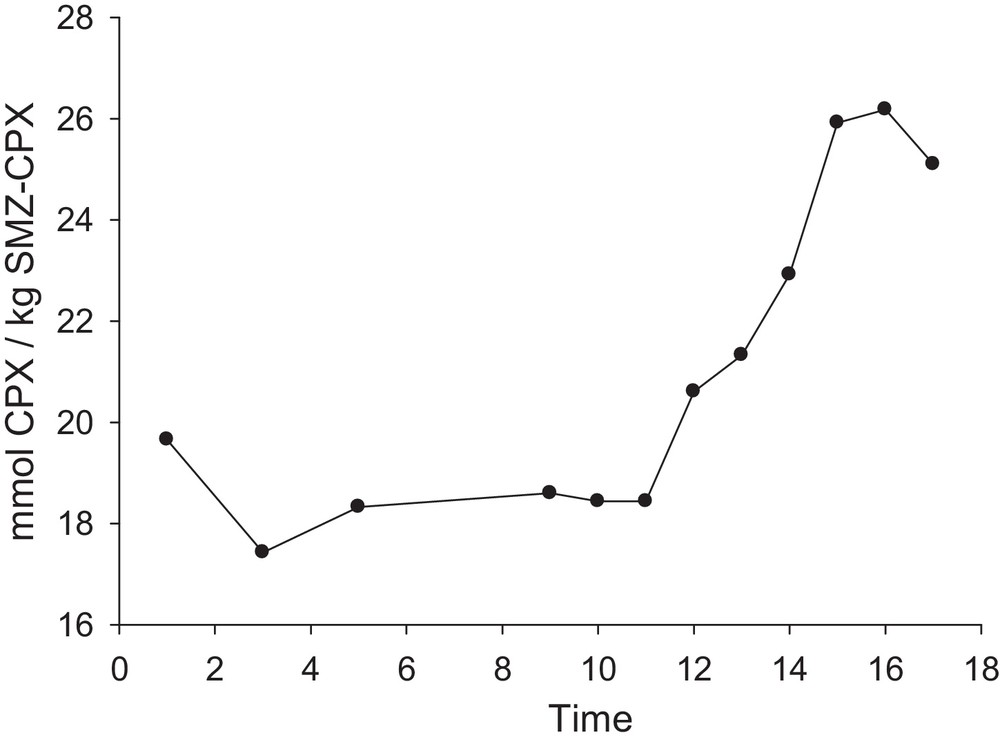
Amount of cephalexin released from unit mass of SMZ–CPX as a function of time (t1 = 3 h, t2 = 5 h, t3 = 9 h, t4 = 10 h, t5 = 11 h, t6= 13 h, t7 = 14 h, t8 = 15 h, t9= 16 h, t10 = 17 h, t11 = 18 h); pH = 2; 2 g SMZ–CPX L−1.
3.3.3 Effect of the presence of different ions on the desorption extent of the drug
Transition metal ions cause an increase in the rate of hydrolysis of β-lactam [63]. Hence, the effects of the type and also the concentration of some cations on the delivery extent of cephalexin were studied; the results are presented in Fig. 13. As shown, as the concentration of Fe2+, Fe3+, K+ and Mg2+ decreases, the amount of released drug diminishes, whereas it is completely inverse for Na+ and Ca2+. It can be linked to their ability to form a complex and also to the pH of the salts solutions. It seems that the molecular model of cephalosporin indicates the coordination between the carbonyl O-atom and the carboxylate group, as shown in Fig. 14 [63]. According to the literature [64], the stability constants of the metal complexes with cephalexin agree with the decrease in the charge density of the metal ions. This behavior can be interpreted based on the bidentate nature of glycine. In this case, the coordination through the α-amino nitrogen and the carboxylic oxygen atoms forms stable five-membered chelate rings, while the cephalosporin ligands may be coordinated through the carboxylate group, the 8-carbonyl group, and the amino nitrogen atom, thus leading to the formation of six-membered rings.
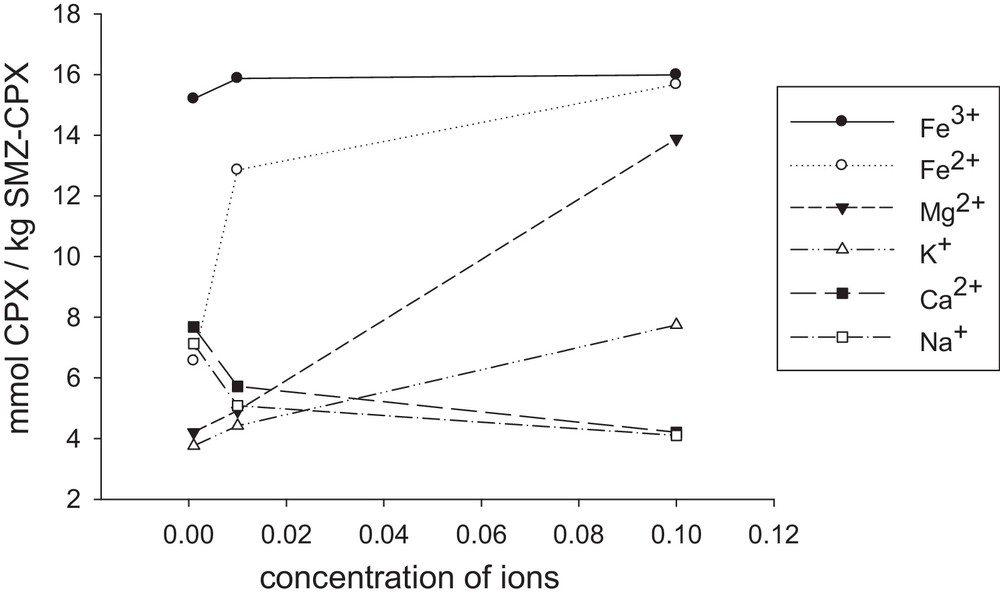
Amount of cephalexin released from a unit mass of SMZ–CPX as a function of the concentration of Fe3+ (a), Fe2+ (b), Mg2+ (c), K+ (d), Ca2+(e), Na+(f), pH = 2; time = 17 h; 2 g SMZ–CPX L−1.
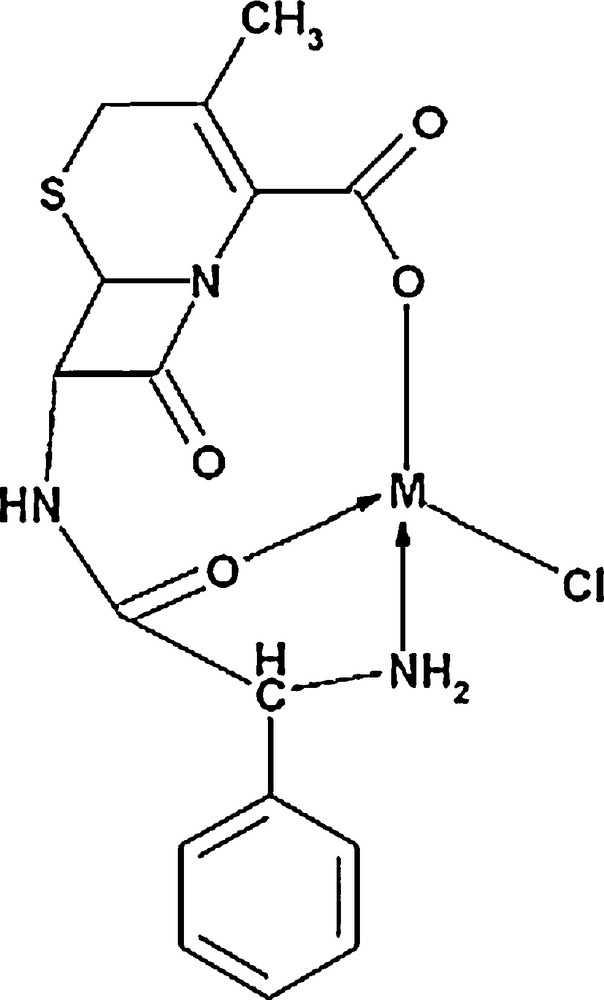
Schematic diagram of the cephalexin metal complexes.
It has been reported that 3d metal cations prefer five-membered to six-membered rings in their chelates [64]. The α-amino group presents C-7 substitution and the carboxylic group at C-3 or the participation of carboxylic group at C-3 and bridge head nitrogen between dehydrothiazine and β-lactam, forming a six-membered metal chelate. These complexes have shown a square planar or octahedral geometry [65]. Considering the above points, this result can be justified on the basis of hard and soft (Lewis) acids and bases (HSAB). According to the Pearson acid base concept, the stability of compounds and also the reaction mechanisms are considered by HSAB. Small species that have high charge states (the charge criterion applies mainly to acids, and to a lesser extent to bases) and weakly polarizable are hard species, while large species that have low charge states and are strongly polarizable are called soft species [66–68]. According to these comments, iron and magnesium cations are hard cations and form stronger complexes with the oxygen atoms of cephlexin. This causes to desorb higher amounts of the adsorbed cephalexin from the SMZ surface.
3.3.4 A comparison with other CPX delivery systems
At the end of manuscript, we decide to collect the results of some CPX delivery systems. Bioavailability and gastric residence time of cephalexin by a hydrodynamically balanced system was studied via gastro retentive dosage forms (GRDFs) method using the direct compression method with different polymers and sodium bicarbonate. Based on the results of the dissolution profiles, the antimicrobial action of cephalexin may be increased in the stomach due to increased retention time and absorption by using HPMC K100 M rather than other formulations; as the concentration of the polymers is increased, a decrease in the drug release rates was observed [69].
Encapsulation of CPX in microspheres, such as CPX-loaded poly (dl-lactide-co-glycolide) (PLGA) with sizes between 2 and 8 μm has been studied [70–73]. In the preparation of PLGA microspheres, using organic solvents, such as chloroform, dichloromethane, acetone, ethyl acetate, glacial acetic acid, and methanol is an unecofriendly technique. CPX is slightly soluble in water (10 mg/ml) and insoluble in the organic phase in which the PLGA was dissolved. As a result, CPX dispersed in the polymer solution will be extracted by the external aqueous phase. However, taken together, the entrapment efficiency of CPX using the single emulsion preparation was too low for practical applications: CPX was found back almost quantitatively (∼98%) in the polyvinyl alcohol phase. Based on the results, the single emulsion technique is not suitable for the preparation of CPX-loaded PLGA microspheres [73]. The size of the microspheres, which significantly affects the efficiency of the method, significantly depends on the type and the ratio of the used organic solvent. In the mentioned work [73], the entrapment of CPX was only 18%.
The potential use of magnetite nanoparticles (MNPs), which were coated with different polymeric materials, such as oleic acid (OA) and pluronic for brain drug targeting purposes, has been studied [74] to control the release of cephalexin. The amount of cephalexin loading in nanoparticles was around 43% and drug release determinations showed that the release of about 90% of the loaded drug took place within 6 h. Drug release during 1 to 360 min from the nanoparticle carrier was comparatively rapid, but followed by a slower rate of release. This could be explained by the change in the carrier's chemical environment experienced by the nanoparticle carrier.
The usefulness of a pH sensitive protein-based hydrogel for in vitro controlled release of cephalexin has been studied via the graft co-polymerization method for the preparation of hydrogels. The synthesized hydrogels were subjected to equilibrium swelling studies in simulated gastric and intestinal fluids (SGF and SIF). The loading drug yield was found to depend on the incubation time, the amount of encapsulated drug and the crosslinker concentration. In vitro drug release studies in different buffer solutions showed that the most important parameter affecting the drug release behavior of hydrogels is the pH of the solution, so, the release rate of cephalexin from hydrogel at pH 7.4 was faster than that at pH 1.2 due to the shrinkage of the hydrogel at pH 1.2 and also to the electrostatic repulsion between the carboxylate groups [75]. The swelling capacity of the hydrogels is affected by the crosslinker concentration and the monomer ratio, so that swelling is decreased by increasing the crosslinker's concentration. The superabsorbent hydrogels exhibited high sensitivity to pH, so that several swelling changes of the hydrogel were observed in pH variations of a wide range (1–13). Ionic repulsion between charged groups incorporated in the gel matrix by an external pH modulation could be assumed as the main driving force responsible for such abrupt swelling changes.
The central nervous system (CNS) is protected by barriers that control the entry of hydrophilic therapeutic agents, such as antibiotics, anticancer agents, or newly developed neuropeptides, into the brain [76]. A number of attempts have been made to overcome this barrier, including the osmotic opening of tight junctions, the use of prodrugs or carrier systems, such as antibodies, liposomes, and nanoparticles [77]. Among the various non-invasive approaches, nanoparticulate carriers and particularly, polymeric nanoparticles, seemed to be among the most interesting methods, by which drugs can be loaded into nanoparticles, adsorbed or chemically linked to their surface. These carriers possess a higher stability in biological fluids and against the enzymatic metabolism than other colloidal ones, such as the liposomes or lipidic vesicles [77,78]. The use of clinoptilolite nanoparticles in our work can be useful for this purpose. The disadvantages of some of the above systems and also the advantages of zeolitic delivery systems are stated in the introductory section.
4 Conclusions
The results of this study showed that the HDTMA surfactant-modified nano-clinoptilolite are a better adsorbent for cephalexin than the raw nano-clinoptilolite sample. This confirms that simple surface adsorption is responsible for drug adsorption onto the raw sample, while electrostatic and also hydrophobic forces play a considerable role in the modified sample. Hence, choosing to optimize the key operating factors, including the concentration of cephalexin, the amount of surfactant per gram of zeolite, the contact time and the pH of the solution, are important factors to reach the highest adsorbed cephalexin extent at shorter times. Also, the results of delivery experiments show that the released drug extent depends on time, pH, and also the diet. The largest amount of the drug is released from the SMZ–CPX system in about 16 h and at pH = 2; the presence of other ions can also affect that.