1 Introduction
To reach an excellent degree of catalyst reusability, during recent years immobilization of homogeneous catalysts on various heterogeneous supports has been used as recyclable catalysts in organic synthesis [1,2]. However, many supports such as SBA-15 [3], MCM-41 [4], TiO2 nanoparticles [5], and iron oxide [6,7] have been used for catalyst supports; the preparation of these supports requires high temperature for calcination or inert atmosphere and also a lot of time and tedious conditions. It is worth mentioning that other heterogeneous supports such as heteropolyacids [8], carbon nanotubes [9], ionic liquids [10,11], graphene oxide [12], or some polymers [13,14] are expensive. Boehmite nanoparticles (γ-AlOOH) are not air or moisture sensitive; therefore, they were prepared in water at room temperature using inexpensive and available materials [15]. Nanoboehmite has several attractive features, such as thermal, chemical, and mechanical stability, high specific surface area (>120 m2/g), easy and ready availability, nontoxicity, ease of surface modification, and favorable biocompatibility [16–18]. In this regard, the surface of boehmite (aluminum oxyhydroxide) nanoparticles, which are covered with high density of hydroxyl groups, can be modified using other functional groups to immobilize homogeneous catalysts [19,20]. Although modification of boehmite has been rarely reported as a heterogeneous support [17–20]. Herein, Br3-TEDETA@Boehmite (TEDETA, N,N,N′,N′-tetraethyldiethylenetriamine) has been reported as a new metal-free catalyst for the synthesis of 2,3-dihydroquinazolin-4(1H)-one and polyhydroquinoline derivatives and also for the selective oxidation of sulfides to sulfoxides. Sulfoxides are valuable synthetic intermediates for the production of chemically and biologically active molecules including therapeutic agents such as antiulcer, antifungal, antibacterial, antiatherosclerotic, antihypertensive, and psychotropic and vasodilators [21–24]. Furthermore, allicin, sulindac, modafinil, garlicnin B-2, garlicnin L-1, and omeprazole are several typical examples of the extensive application of these intermediates in the pharmaceutical and fine chemical industries [22,25]. In addition, sulfoxides are also valuable in the CC bond formation and molecular rearrangements [26]. Besides, 2,3-dihydroquinazolin-4(1H)-one and polyhydroquinoline derivatives have been reported to possess a wide range of biological properties and pharmaceutical activities [27,28]. For example, altanserin and nitroaltanserin are drugs for 5-HT2A receptor antagonists [29], also 2-(2-hydroxyphenyl)-4(3H)-quinazolinone was used in the detection of metal ions [30]. Furthermore, 2,3-dihydro-4(1H)-quinazolinones are starting materials for the preparation of 4-(3H)-quinazolinones [31]. The novelty of this work is that it is for the first time that an ion has been grafted on boehmite nanoparticles, which have been rarely used as catalyst supports. In addition, synthesis of 2,3-dihydroquinazolin-4(1H)-one and polyhydroquinoline derivatives has been commonly reported in the presence of Louise or protonic acid [39–41]. In this study, tribromide ions supported on boehmite nanoparticles were used as catalysts for the synthesis of 2,3-dihydroquinazolin-4(1H)-one and polyhydroquinoline derivatives. Furthermore, Br3-TEDETA@Boehmite is a selective catalyst for the sulfoxidation reaction.
2 Result and discussion
2.1 Catalyst preparation
Br3-TEDETA@Boehmite was prepared by the concise route outlined in Scheme 1. Modified boehmite nanoparticles were prepared according to the new reported procedure [15].

Preparation process of Br3-TEDETA@Boehmite.
2.2 Catalyst characterization
The mentioned nanocatalyst has been characterized by thermogravimetric analysis/differential thermal analysis (TGA/DTA), Brunauer–Emmett–Teller (BET), X-ray diffraction (XRD), energy-dispersive X-ray spectroscopy (EDS), scanning electron microscopy (SEM), and Fourier transform infrared (FT-IR) spectroscopy techniques.
The size of particles was evaluated by SEM technique. The several SEM images of Br3-TEDETA@Boehmite are shown in Fig. 1. These SEM images illustrated that the particles of the catalyst were formed as nanometer-sized particles of about 20–60 nm (Fig. 1). To indicate the content of element type in catalyst, EDS analysis of Br3-TEDETA@Boehmite was performed (Fig. 2). As depicted, the EDS spectrum of catalyst shows the presence of Al, Si, O, C, N, and Br species in the catalyst.

SEM images of Br3-TEDETA@Boehmite.
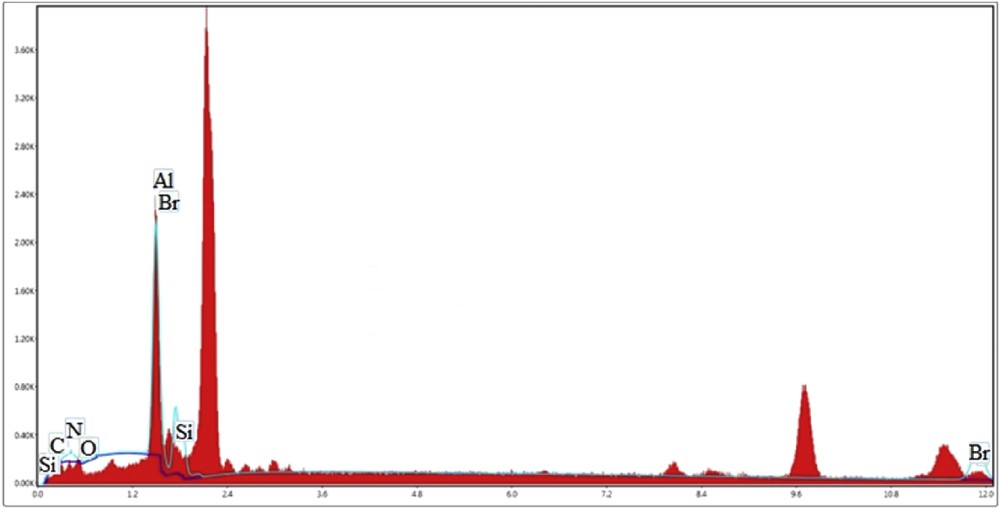
EDX spectrum of Br3-TEDETA@Boehmite.
The FT-IR spectra of boehmite nanoparticles, nPr-Cl@boehmite, TEDETA@Boehmite, and Br3-TEDETA@Boehmite are shown in Fig. 3. Two bands at 3086 and 3308 cm−1 indicated the hydroxyl groups on the surface of boehmite nanoparticles [32]. Several peaks in FT-IR spectra at 480, 605 and 735 cm−1 can be attributed to the AlO bonds [20]. Also, one band at 1650 cm−1 and two bands at 1164 and 1069 cm−1 correspond to the nitrate impurity vibration and hydrogen bands of OH…OH surface, respectively [32]. In the FT-IR spectra of TEDETA@Boehmite, OSi and CH stretching vibrations appear at 1070 and 2930 cm−1, respectively [1].
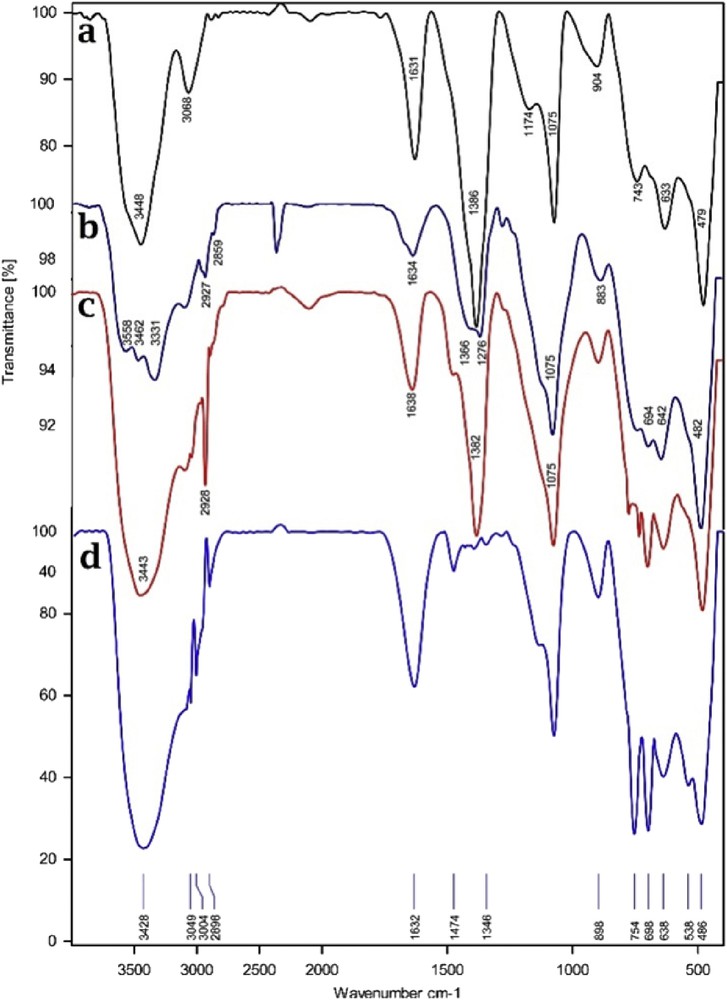
FT-IR spectra of boehmite nanoparticles (a), nPr-Cl@boehmite (b), TEDETA@Boehmite (c), and Br3-TEDETA@Boehmite (d).
The TGA/DTA was used to determine the percent of organic functional groups, which were immobilized on the surface of boehmite nanoparticles. Fig. 4 shows the TGA curves for boehmite nanoparticles and catalyst. The weight loss at temperatures <200 °C (about 10%) is caused by the removal of the adsorbed solvents [20,32]. In the TGA/DTA profile of the catalyst, organic layers have been desorbed at temperatures >350 °C (about 29%).
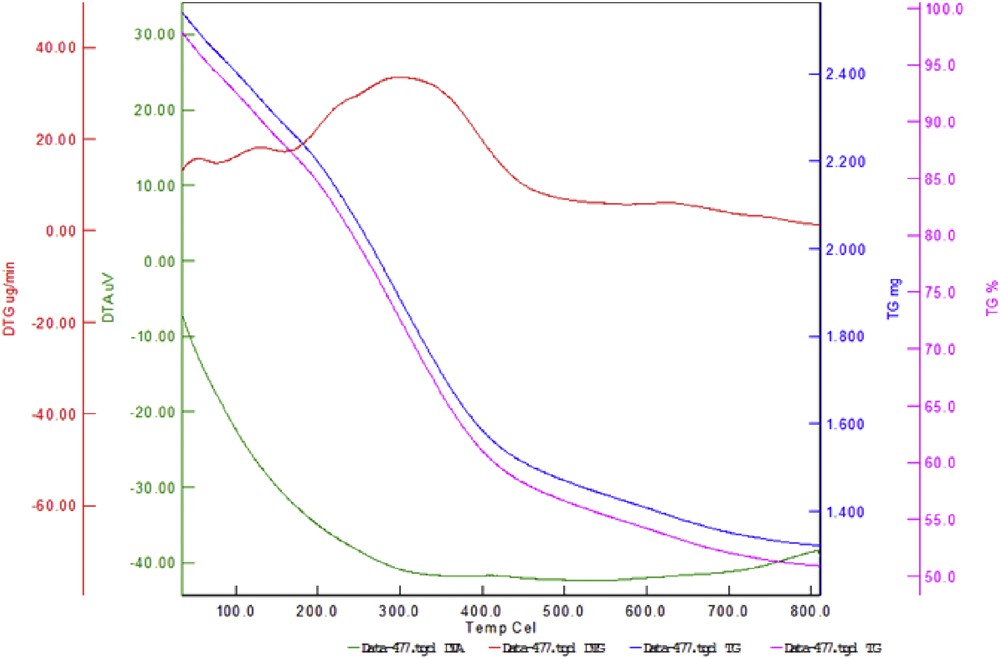
TGA/DTA diagram of Br3-TEDETA@Boehmite.
The XRD patterns of Br3-TEDETA@Boehmite are shown in Fig. 5. As it has been clearly illustrated, XRD pattern of Br3-TEDETA@Boehmite shows a good agreement with standard XRD pattern of boehmite nanoparticles in which all of the peaks confirmed the crystallization of boehmite with an orthorhombic unit cell [15,18]. This agreement is strong evidence that boehmite phase was stable after modification.

XRD pattern of Br3-TEDETA@Boehmite.
The nitrogen adsorption–desorption isotherms of Br3-TEDETA@Boehmite are shown in Fig. 6. On the basis of the IUPAC classification, these materials display a typical IV isotherm, which can be regarded as the characteristic of mesoporous materials. On the basis of the BET technique, the surface area for Br3-TEDETA@Boehmite, which is 37.6 m2/g, is lower than the surface area of boehmite nanoparticles (122.8 m2/g) [32] because of the immobilization of organic layers and the catalyst on the surface of boehmite nanoparticles.
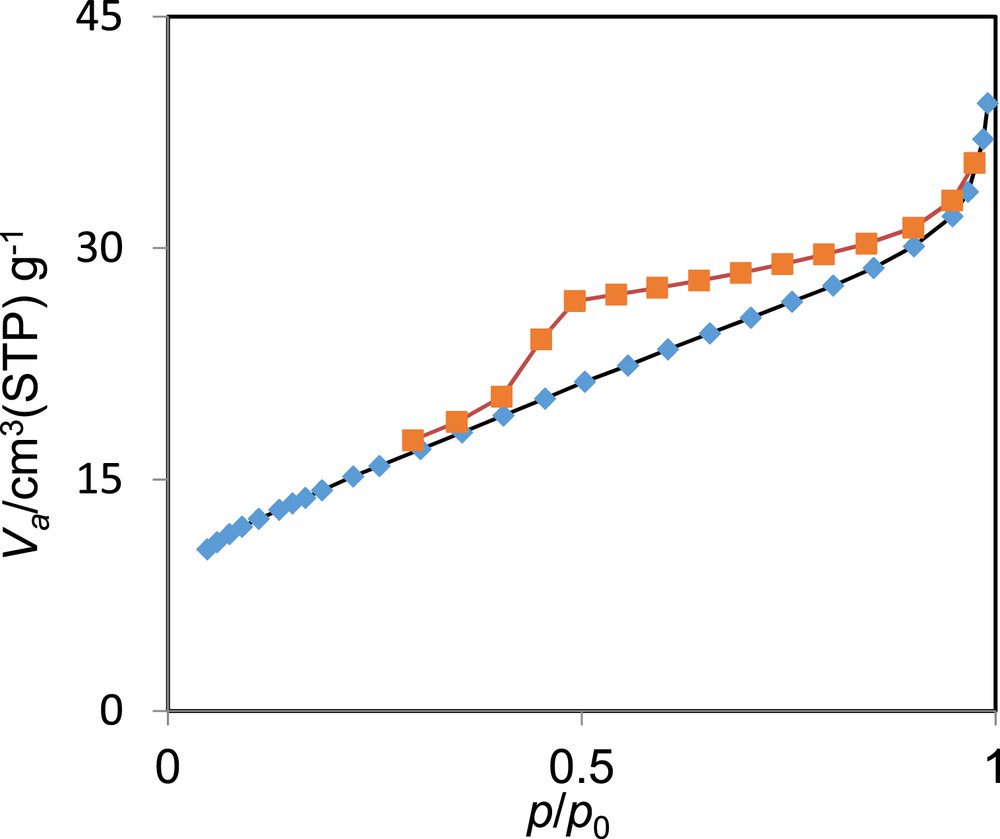
Nitrogen adsorption–desorption isotherms of Br3-TEDETA@Boehmite.
2.3 Catalytic study
To study the catalytic activity of Br3-TEDETA@Boehmite, we were interested in finding a simple and efficient procedure for the synthesis of 2,3-dihydroquinazolin-4(1H)-one derivatives using condensation of aldehydes and anthranilamide in the presence of Br3-TEDETA@Boehmite as a recoverable nanocatalyst (Scheme 2).

Br3-TEDETA@Boehmite catalyzed the synthesis of 2,3-dihydroquinazolin-4(1H)-one derivatives.
The reaction conditions for the synthesis of 2,3-dihydroquinazolin-4(1H)-one derivatives were optimized in the condensation of 4-chlorobenzaldehyde with ammonium acetate, dimedone, and ethyl acetoacetate in the presence of different amounts of Br3-TEDETA@Boehmite in various solvents as a model reaction (Table 1). The sample reaction cannot proceed in the absence of Br3-TEDETA@Boehmite even after 3 h (Table 1, entry 8). Although increasing the amount of catalyst from 30 mg (Table 1, entry 6) to 40 mg (Table 1, entry 5) led to an increase in the yield of the product from 50% to 96%, increasing the amount of catalyst (from 40 to 50 mg) had no significant effect on the yield of product or reaction time. The model reaction was also carried out in the presence of a little amount of the catalyst (Table 1, entries 6 and 7), which caused low yields of the products. To find the best reaction conditions, different temperatures were examined for the model reaction. As shown in Table 1, best results were obtained at 70 °C. In this sense, it is worth noting that when the model reaction was tested at 50 °C, the reaction yield decreased to 63% (Table 1, entry 9). Among the different solvents such as PEG, ethyl acetate, DMSO, and H2O, the best results were obtained in ethyl acetate.
Optimization of the reaction conditions for the synthesis of 2,3-dihydroquinazolin-4(1H)-ones in the condensation of 4-chlorobenzaldehyde with anthranilamide.
Entry | Solvent | Catalyst (mg) | Time (min) | Temperature (°C) | Yield (%)a |
1 | H2O | 40 | 35 min | 70 | 20 |
2 | PEG | 40 | 35 min | 70 | 25 |
3 | DMSO | 40 | 35 min | 70 | 45 |
4 | Ethyl acetate | 50 | 35 min | 70 | 97 |
5 | Ethyl acetate | 40 | 35 min | 70 | 96 |
6 | Ethyl acetate | 30 | 90 min | 70 | 50 |
7 | Ethyl acetate | 20 | 90 min | 70 | 39 |
8 | Ethyl acetate | – | 3 h | 70 | Trace |
9 | Ethyl acetate | 40 | 35 min | 50 | 63 |
a Isolated yields.
As shown in Table 1, 40 mg of Br3-TEDETA@Boehmite in ethyl acetate at 70 °C was found to be the best reaction conditions for the synthesis of 2,3-dihydroquinazolin-4(1H)-ones.
To demonstrate the generality and efficiency of this method, different aldehydes were tested under optimized reaction conditions (Table 2). As shown in Table 2, the catalytic system worked exceedingly well in the synthesis of 2,3-dihydroquinazolin-4(1H)-ones with a wide range of functional groups. The pure products were obtained in short reaction times and high yields.
Synthesis of 2,3-dihydroquinazolin-4(1H)-ones catalyzed by Br3-TEDETA@Boehmite.
Entry | Aldehyde | Product | Time (min) | Yield (%)a | Melting point (°C) | Reported melting point [reference] |
1 | Image 1 | Image 2 | 35 | 96 | 193–195 | 193–194 [33] |
2 | Image 3 | Image 4 | 60 | 94 | 204–206 | 206–208 [33] |
3 | Image 5 | Image 6 | 60 | 91 | 195–197 | 190–192 [34] |
4 | Image 7 | Image 8 | 105 | 93 | 268–270 | 278–280 [35] |
5 | Image 9 | Image 10 | 90 | 85 | 183–185 | 182–183 [36] |
6 | Image 11 | Image 12 | 160 | 87 | 196–198 | 193–195 [33] |
7 | Image 13 | Image 14 | 70 | 86 | 207–209 | 210–212 [37] |
8 | Image 15 | Image 16 | 80 | 87 | 219–221 | 220–222 [37] |
9 | Image 17 | Image 18 | 90 | 89 | 190–191 | 188–190 [27] |
10 | Image 19 | Image 20 | 40 | 91 | 163–165 | 165–167 [38] |
11 | Image 21 | Image 22 | 135 | 88 | 197–199 | 197–199 [39] |
12 | Image 23 | Image 24 | 90 | 91 | 218–220 | 219–220 [33] |
13 | Image 25 | Image 26 | 70 | 92 | 201–203 | 201–202 [37] |
14 | Image 27 | Image 28 | 275 | 86 | 195–197 | 200–201 [37] |
15 | Image 29 | Image 30 | 170 | 90 | 167–169 | 166–169 [33] |
16 | Image 31 | Image 32 | 270 | 92 | 171–174 | 174–176 [40] |
17 | Image 33 | Image 34 | 270 | 93 | 176–178 | 174–176 [37] |
a Isolated yield.
In the second phase of our study, the catalytic activity of Br3-TEDETA@Boehmite in the one-pot synthesis of polyhydroquinoline derivatives (Scheme 3) was reported.

Br3-TEDETA@Boehmite catalyzed the synthesis of polyhydroquinoline derivatives.
To find the best reaction conditions, various parameters such as different amounts of catalyst and various solvents were investigated in the condensation of 4-chlorobenzaldehyde, dimedone, ethyl acetoacetate, and ammonium acetate as a model reaction (Table 3). Initially, the amount of catalyst was optimized and, as a result, the best results were obtained with 0.04 g of Br3-TEDETA@Boehmite (Table 3, entry 4). Moreover, the experiments show that the reaction does not occur in the absence of the catalyst (Table 3, entry 7). As shown in Table 3, increase in the amount of the catalyst led to an increase in the yield of obtained products. Meanwhile, the best results were obtained in the presence of 40 mg of catalyst. When the amount of catalyst was increased to 50 mg (Table 3, entry 3), no significant change in the yield of product or reaction time was observed. Consequently, the effect of different solvents was examined (Table 3, entries 1–3). Among the various solvents, the highest yield has been obtained in ethanol with short reaction time. Finally, the effect of temperature on the model reaction was studied and best results were obtained at 80 °C (Table 3, entry 4).
Optimization of reaction conditions for the synthesis of polyhydroquinoline for condensation of 4-chlorobenzaldehyde with dimedone, ethyl acetoacetate, and ammonium acetate.
Entry | Solvent | Catalyst (mg) | Time (min) | Temperature (°C) | Yield (%)a |
1 | Ethyl acetate | 40 | 75 | 80 | 84 |
2 | PEG | 40 | 75 | 80 | 55 |
3 | Ethanol | 50 | 75 | 80 | 95 |
4 | Ethanol | 40 | 75 | 80 | 93 |
5 | Ethanol | 30 | 100 | 80 | 75 |
6 | Ethanol | 20 | 100 | 80 | 70 |
7 | Ethanol | – | 100 | 80 | –b |
8 | Ethanol | 40 | 75 | 60 | 44 |
9 | Ethanol | 40 | 75 | 40 | 40 |
a Isolated yield.
b No reaction.
To explore the activity of these catalysts, various aldehydes, including a wide range of electron-donating and electron-withdrawing (Table 4) functional groups, have been described in optimum conditions. Besides, the corresponding polyhydroquinoline compounds were obtained in good to excellent yields. As a point of note, 1,4-benzenedicarboxaldehyde was converted to bis-polyhydroquinoline in 90% of yield (Table 4, entry 14).
Synthesis of polyhydroquinoline derivatives catalyzed by Br3-TEDETA@Boehmite.
Entry | Aldehyde | Product | Time (min) | Yield (%)a | Melting point (°C) | Reported melting point [reference] |
1 | Image 35 | Image 36 | 400 | 93 | 214–216 | 216–218 [41] |
2 | Image 37 | Image 38 | 75 | 96 | 228–230 | 233–235 [42] |
3 | Image 39 | Image 40 | 75 | 98 | 253–257 | 254–256 [41] |
4 | Image 41 | Image 42 | 340 | 87 | 234–237 | 239–241 [43] |
5 | Image 43 | Image 44 | 500 | 85 | 178–180 | 178–180 [44] |
6 | Image 45 | Image 46 | 30 | 90 | 187–190 | 183–185 [27] |
7 | Image 47 | Image 48 | 560 | 96 | 246–248 | 247–249 [36] |
8 | Image 49 | Image 50 | 90 | 89 | 192–194 | – |
9 | Image 51 | Image 52 | 240 | 88 | 248–250 | 247–248 [41] |
10 | Image 53 | Image 54 | 180 | 92 | 202–204 | 201–202 [36] |
11 | Image 55 | Image 56 | 360 | 92 | 169–171 | 172–174 [27] |
12 | Image 57 | Image 58 | 330 | 87 | 231–234 | 229–234 [45] |
13 | Image 59 | Image 60 | 360 | 88 | 234–236 | 238–241 [46] |
14 | Image 61 | Image 62 | 210 | 93 | 157–159 | – |
15 | Image 63 | Image 64 | 200 | 90 | 194–195 | 202–204 [47] |
a Isolated yields.
Finally, the oxidation of sulfides to sulfoxides has been examined using H2O2 in the presence of these nanomaterials (Scheme 4).

Br3-TEDETA@Boehmite catalyzed the oxidation of sulfides to sulfoxides.
To optimize the reaction conditions, we selected the oxidation of methyl phenyl sulfide using H2O2 in various solvents and in the presence of different amounts of the catalyst (Table 5). Different amounts of the catalyst were examined and the best results were obtained using 0.04 g of the catalyst (Table 5, entry 4). As shown in Table 5, increase in the amount of the catalyst (from 0.04 to 0.05 g) did not cause a significant change in reaction time or yield. Also, amount of hydrogen peroxide needed in the model reaction was studied and 0.4 mL of hydrogen peroxide was selected for oxidation sulfides to sulfoxides (Table 5, entry 4). Decreasing the amount of hydrogen peroxide from 0.4 mL (Table 5, entry 4) to 0.3 mL (Table 5, entry 8) led to decrease in yield of the product from 88% to 53% even during long reaction times. Moreover, to find the best reaction conditions, various solvents and solvent-free conditions were examined in the model reaction and the best results were obtained in ethyl acetate. As shown in Table 5, methyl phenyl sulfide (1 mmol) in the presence of Br3-TEDETA@Boehmite (0.04 g) and ethyl acetate at room temperature was found to be the ideal reaction conditions for the formation of methyl phenyl sulfoxide.
Optimization of the reaction conditions for oxidation of methyl phenyl sulfide as a model substrate.
Entry | Solvent | Catalyst (mg) | H2O2 (mL) | Time (min) | Yield (%)a |
1 | Acetonitrile | 40 | 0.4 | 90 | 75 |
2 | Ethanol | 40 | 0.4 | 100 | 79 |
3 | Solvent-free | 40 | 0.4 | 90 | 46 |
4 | Ethyl acetate | 40 | 0.4 | 60 | 88 |
5 | Ethyl acetate | 20 | 0.4 | 80 | 75 |
6 | Ethyl acetate | 50 | 0.4 | 80 | 90 |
7 | Ethyl acetate | 60 | 0.4 | 20 | 93 |
8 | Ethyl acetate | 40 | 0.3 | 100 | 53 |
a Isolated yield.
To explore the catalytic activity of Br3-TEDETA@Boehmite, various sulfides, including aromatic and aliphatic sulfides, were converted to their corresponding sulfoxides in the presence of Br3-TEDETA@Boehmite under optimized conditions as all of the products were obtained with high to excellent yields (Table 6). To show the chemoselectivity of this protocol, sulfides, including other functional groups such as hydroxyl groups or olefin, were subjected to the oxidation reaction. These functional groups remained intact during the conversion of sulfides into sulfoxides (Scheme 5). Also, because of mild conditions of the described heterogeneous system, overoxidation to sulfone after oxidation of sulfides to sulfoxide was not observed.
Synthesis of polyhydroquinoline derivatives catalyzed by Br3-TEDETA@Boehmite.
Entry | Sulfide | Product | Time (min) | Yield (%)a | Melting point (°C) | Reported melting point [reference] |
1 | Image 65 | Image 66 | 20 | 93 | Oil | Oil [48] |
2 | Image 67 | Image 68 | 25 | 90 | 130–132 | 131–133 [49] |
3 | Image 69 | Image 70 | 20 | 80 | Oil | Oil [50] |
4 | Image 71 | Image 72 | 25 | 83 | Oil | Oil [49] |
5 | Image 73 | Image 74 | 40 | 91 | Oil | Oil [55] |
6 | Image 75 | Image 76 | 35 | 85 | Oil | Oil [48] |
7 | Image 77 | Image 78 | 45 | 80 | Oil | Oil [49] |
8 | Image 79 | Image 80 | 40 | 94 | 59–62 | 61–64 [57] |
a Isolated yields.

Chemoselective oxidation of sulfides in the presence of other functional groups using Br3-TEDETA@Boehmite as a catalyst.
2.4 Recyclability of the catalyst
Regarding the aforementioned statements, we examined the recycling of Br3-TEDETA@Boehmite for the synthesis of 2,3-dihydroquinazolin-4(1H)-one and polyhydroquinoline derivatives using 4-chlorobenzaldehyde as the model compound. After completion of the reaction, the catalyst was separated from the product. The remaining catalyst was washed with ethyl acetate and subjected to the next run. The recovered catalyst was reused up to five runs without any significant loss of its catalytic activity (Fig. 7).
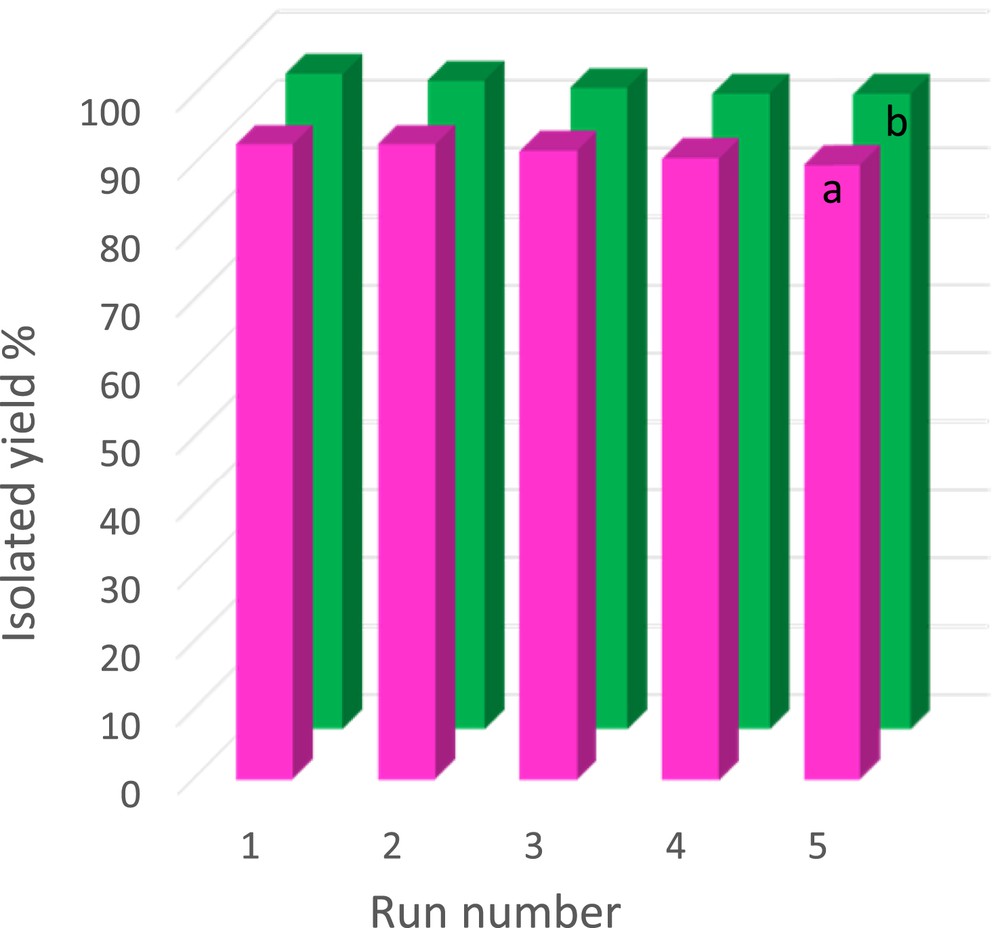
The recycling experiment of Br3-TEDETA@Boehmite in the synthesis of polyhydroquinoline (a) and 2,3-dihydroquinazolin-4(1H)-one (b) using 4-chlorobenzaldehyde as a model substrate.
Besides, the recycled catalyst has been characterized by FT-IR spectroscopy technique. FT-IR spectra of the recycled catalyst are shown in Fig. 8. As illustrated in Fig. 8, FT-IR spectrum of recycled Br3-TEDETA@Boehmite (Fig. 8) shows a very good agreement with FT-IR spectrum of fresh Br3-TEDETA@Boehmite (Fig. 3d). Stability of Br3-TEDETA@Boehmite after recycling was confirmed by position of stretching vibrations in FT-IR spectra of the fresh and recycled catalyst. This agreement is strong evidence for the high stability of Br3-TEDETA@Boehmite after being recycled.
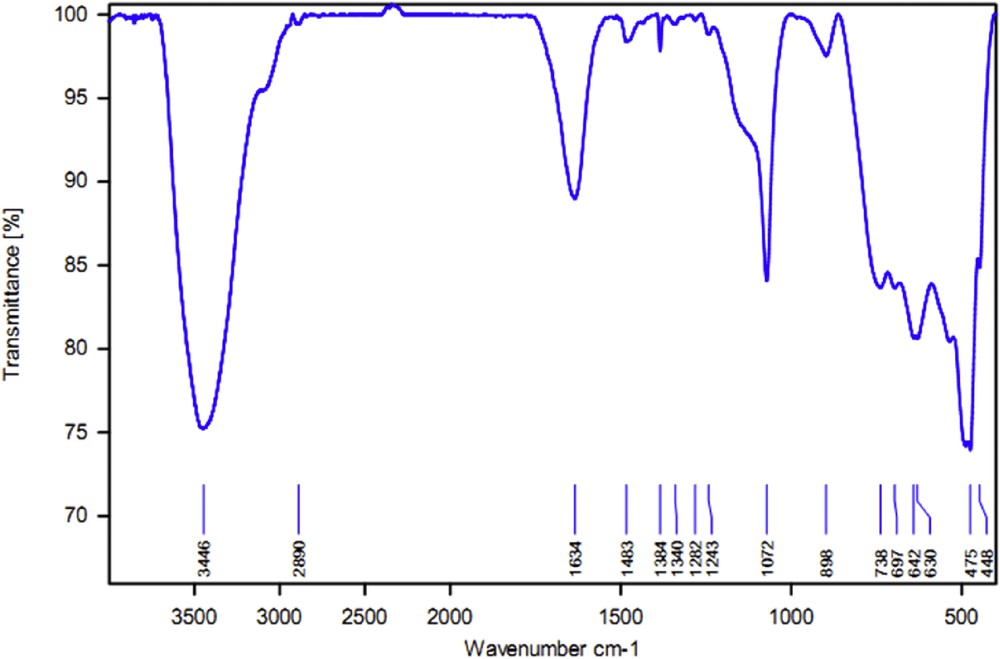
FT-IR spectra of recycled Br3-TEDETA@Boehmite.
2.5 Comparison of the catalyst
The efficiency of Br3-TEDETA@Boehmite was demonstrated by comparing the obtained results in the present work with previous methods for synthesis of 2,3-dihydroquinazolin-4(1H)-one derivatives using 4-chlorobenzaldehyde and oxidation of methyl phenyl sulfide (Table 7). As shown, better reaction times and higher yields were obtained using Br3-TEDETA@Boehmite as compared with other reported catalysts. In addition, Br3-TEDETA@Boehmite can be reused for several runs.
Comparison of Br3-TEDETA@Boehmite for the synthesis of 2,3-dihydroquinazolin-4(1H)-ones using 4-chlorobenzaldehyde and oxidation of methyl phenyl sulfide with previously reported procedures.
Entry | Substrate | Catalyst | Time (min) | Yielda (%) [reference] |
1 | Ph-SCH3 | Ni-SMTU@Boehmite | 75 | 97 [50] |
2 | Ph-SCH3 | Polymer-anchored Cu(II) | 180 | 90 [51] |
3 | Ph-SCH3 | Cu-SPATB/Fe3O4 | 95 | 98 [52] |
4 | Ph-SCH3 | Cd-salen-MCM-41 | 150 | 98 [53] |
5 | Ph-SCH3 | TsOH | 240 | 88 [54] |
6 | Ph-SCH3 | Ni-salen-MCM-41 | 156 | 95 [53] |
7 | Ph-SCH3 | DSA@MNPs | 360 | 98 [55] |
8 | Ph-SCH3 | VO2F(dmpz)2 | 300 | 95 [56] |
9 | Ph-SCH3 | Br3Fe3O4 | 50 | 98 [57] |
10 | Ph-SCH3 | Br3-TEDETA@Boehmite | 20 | 93 [this work] |
11 | 4-ClC6H4CHO | GSA@MNPs | 50 | 99 [39] |
12 | 4-ClC6H4CHO | [Bmim]PF6 | 40 | 90 [58] |
13 | 4-ClC6H4CHO | TBAB/100 °C | 60 | 75 [59] |
14 | 4-ClC6H4CHO | SiO2FeCl3 | 420 | 87 [60] |
15 | 4-ClC6H4CHO | 2-(N-morpholino)ethanesulfonic acid | 180 | 89 [61] |
16 | 4-ClC6H4CHO | ZrCl4 | 37 | 91 [62] |
17 | 4-ClC6H4CHO | [NMP][H2PO4] | 38 | 79 [63] |
18 | 4-ClC6H4CHO | Boehmite-SSA | 50 | 98 [27] |
19 | 4-ClC6H4CHO | Pd-SBTU@Fe3O4 | 140 | 95 [41] |
20 | 4-ClC6H4CHO | Br3-TEDETA@Boehmite | 35 | 96 [this work] |
a Isolated yield.
3 Experimental section
3.1 Materials
The sodium hydroxide, Al(NO3)3·9H2O, 3-chloropropyltrimethoxysilane, TEDETA, solvents, and other chemicals used in the present study were purchased from Merck, Aldrich, or Fluka companies and used without further purification.
3.2 Characterization techniques
Powder XRD of the catalyst was performed with Cu Kα radiation at 40 kV and 30 mA using a PW1730 instrument from Philips Company. FT-IR spectra were recorded with KBr pellets using a VRTEX 70 model Bruker FT-IR spectrometer. The component analysis was carried out by the energy dispersive X-ray spectroscopy (EDS) using an FEI Quanta 200 Scanning Electron Microscope. TGA of the samples was obtained between 30 and 800 °C with heating rate of 10 °C min−1 using Perkin Elmer Pyris Diamond Thermogravimetric Analyzers. The particle size and morphology were performed using an FEI Quanta 200 Scanning Electron Microscope at an accelerating voltage of 25 kV. Melting points of all compounds were determined using Griffin melting point apparatus. Nitrogen adsorption isotherms were determined using a standard gas manifold at 77 K to surface characteristics of the catalyst using a BELSORP MINI II device. Also, the catalyst sample was degassed at 120 °C for 2 h using a BEL PREP VAC II device before analysis.
3.3 Preparation of the catalyst
Initially, modified boehmite nanoparticles using 3-chloropropyltrimethoxysilane (nPr-Cl@Boehmite) were prepared according to the newly reported procedure [17]. Besides, the obtained nPr-Cl@Boehmite (1.0 g) was dispersed in toluene and mixed with 1.5 mL of TEDETA. The mixture was stirred at 90 °C for 24 h. The solid product (TEDETA@Boehmite) was obtained after being washed with ethanol and dried at 50 °C. Then, 1 mL of HBr was added to TEDETA@Boehmite (1 g) and stirred for 1 h at room temperature. Finally, the resultant product (1 g) was dispersed in CCl4 (5 mL). Subsequently, Br2 (2.5 mL) was added to the mixture and stirred for 24 h at room temperature. Then, the final product (Br3-TEDETA@Boehmite) was separated and washed with CCl4 to remove the unattached substrates.
3.4 General procedure for the oxidation of sulfides
A mixture of sulfide (1 mmol), H2O2 (0.4 mL), and Br3-TEDETA@Boehmite (0.06 g) was stirred at room temperature in ethyl acetate, and the progress of the reaction was monitored by TLC (thin-layer chromatography). After completion of the reaction, the catalyst was separated using filtration and washed with ethyl acetate. Accordingly, the product was extracted with ethyl acetate. The organic layer was dried over anhydrous Na2SO4 (1.5 g). Finally, the organic solvents were evaporated, and products were obtained in good to high yield.
3.5 General procedure for the synthesis of polyhydroquinoline derivatives
A mixture of aldehyde (1 mmol), dimedone (1 mmol), ethyl acetoacetate (1 mmol), ammonium acetate (1.2 mmol), and Br3-TEDETA@Boehmite (0.04 g) was stirred in ethanol under reflux conditions, and the progress of the reaction was monitored by TLC. After completion of the reaction, the catalyst was separated and washed with ethyl acetate. Then, the solvent was evaporated and all of the products were recrystallized in ethanol. It is worth mentioning that pure polyhydroquinoline derivatives were obtained in good to excellent yields.
3.6 General procedure for the synthesis of 2,3-dihydroquinazolin-4(1H)-ones derivatives
A mixture of Br3-TEDETA@Boehmite (0.01 g), anthranilamide (1 mmol), and aldehyde (1 mmol) was stirred at 80 °C in ethanol (2 mL). The progress was monitored by TLC. After completion of the reaction, the reaction mixture was cooled to room temperature. Afterward, CH2Cl2 (2 × 5 mL) was added and the catalyst was separated. CH2Cl2 was evaporated under reduced pressure to afford the essentially pure products. Finally, all products were recrystallized in ethanol for further purification.
4 Conclusions
Tribromide ions on boehmite nanoparticles (Br3-TEDETA@Boehmite) were prepared and characterized by FT-IR spectroscopy, TGA, BET, XRD, EDS, and SEM techniques. Br3-TEDETA@Boehmite has been used as a highly efficient and reusable nanocatalyst in the selective oxidation of sulfides and the synthesis of polyhydroquinoline, and 2,3-dihydroquinazolin-4(1H)-one derivatives. All products were obtained in good yields. The reusability of this catalyst was studied. Finally, this catalyst, which is compatible with the environment because of metal-free and nontoxicity, was prepared using inexpensive and available materials.
Acknowledgments
This work was supported by the research facilities of Ilam University, Ilam, Iran.