1. Introduction
Contamination of wastewater due to industrial spills creates an alarming situation, with the presence of numerous pollutants in drinking water leading to undesirable effects on ecosystems and human life [1]. These non-degradable wastes, including heavy metals or toxic organic compounds, result from different industries (fertilizers, metallurgy, leather, mining, galvanizing, pesticides, electrolysis, electro-osmosis, plastics, batteries) and end up in the water directly or indirectly. The effect of these compounds can be toxic, mutagenic, neurotoxic or carcinogenic [2, 3]. The most common heavy metal ions in polluted waters are: Pb(II), Hg(II), Cr(III), Cd(II), Cu(II), Ni(II) and As(III) [4]. According to the World Health Organization [5], the presence of lead in concentrations exceeding the allowed limit (>0.1 mg/L) can affect the nervous system, kidneys, brain, liver and can lead to health problems such as hepatitis, nephritic syndrome, anemia, and so on [6]. Because it has affinity for the thiol (–SH), phosphate (
Various studies have shown that hydrotalcites, including anionic clays and layered double hydroxides (LDHs) are effective materials for adsorption/ removal of heavy metals from aqueous solutions [8, 9]. These are a class of two-dimensional nanostructured compounds, characterized by a stratified lamellar structure similar to brucite [Mg(OH)2] [10]. LDHs are represented by the general formula [
Mg2Al–LDH hydrotalcite was investigated in Pb(II) adsorption process, exhibiting an adsorption capacity of 66.16 mg/g [19]. By substituting Al ions with Fe ions, a much higher capacity (865.36 mg/g) was obtained [20]. The FeMnMg–LDH-type material, obtained by the combined precipitation (co-precipitation) method, was used to remove Pb(II) ions from wastewater, having an adsorption capacity of 421.42 mg/g [13]. MgAl–LDH-type materials intercalated with different amino acids (phenylalanine, tyrosine, serine) showed good adsorption capacity for Pb(II) ions. The highest capacity was obtained for the MgAl–phenylalanine–LDH material (852 mg/g) [21]. Inspired by mussel adhesion protein, Yang et al. [22] have synthesized MgAl–LDH/ carbon fiber film modified by polydopamine for highly efficient removal of Pb2+ (1223.15 mg/g).
The aim of this study was to characterize and determine the maximum sorption capacity of Pb(II) ions for six adsorbents based on MgAl–LDHs, synthesized using co-precipitation route by modifying some of the synthesis parameters (temperature, ageing time) in order to tailor their adsorption properties.
2. Materials and methods
2.1. Reagents and materials
All experiments were performed using analytical grade reagents, without prior purification. Al(NO3)3 ⋅ 9H2O, Mg(NO3)2 ⋅ 6H2O, Na2CO3, NaOH (Aldrich, 99.9%), were used for the LDHs synthesis. The sorption experiments were performed using a 1500 mg⋅L−1 Pb(II) stock solution, prepared by dissolving 2.385 g Pb(NO3)2 in 1000 mL of distilled water. The working solutions with different Pb(II) concentrations were prepared by its dilution. For Flame Atomic Absorption Spectrometry (FAAS) measurements, the calibration curve was recorded in the concentration range 1–20 mg⋅L−1 using a standard Pb(II) solution of 1000 mg⋅L−1. The pH was adjusted in the range 2–6 using 0.1 M HCl or 0.1 M NaOH solutions.
2.2. LDHs synthesis
All the LDHs were prepared by a co-precipitation method similar to that described in [23]. Briefly, MgAl–LDH containing Mg2+ and Al3+ (Mg2+∕Al3+ = 2∕1) as cations in the LDHs layers was prepared by the slow addition of a 1 M aqueous solution of Mg(NO3)2 ⋅ 6H2O and Al(NO3)3 ⋅ 9H2O, to a Na2CO3 (0.5 × 10−2 M) solution under stirring. The synthesis temperatures and the ageing time of the samples were optimized as controlled variable synthesis parameters. The obtained MgAl–LDH is symbolized as PY(X), where X represents the synthesis temperature (X is equal to 5 °C, 15 °C, 30 °C and 55 °C) and Y is the ageing time of the obtained sample (Y = 24 h or 1 h). For all the samples the pH value was kept constant at 9, by adding suitable quantities of 0.1 M NaOH aqueous solution. The obtained precipitates were recovered by filtration, washed several times with distilled water to remove soluble salts and dried at 80 °C under vacuum overnight. Following these procedures, the synthesized MgAl–LDHs were further denoted as P24(5), P24(15), P24(30), P1(30), P24(55) and P1(55).
2.3. Characterization techniques
Scanning electron microscopy (SEM) analyses were performed at 2 kV using a Hitachi SU8010 microscope. The elemental composition was determined by energy dispersive X-ray spectroscopy (EDX) at an acceleration voltage of 15 kV. A Bruker Alpha spectrometer with ZnSe crystal and an attenuated total reflectance (ATR) module was used for the Fourier transform infrared spectroscopy (FTIR) analyses, the spectra being recorded in the spectral range 4000–400 cm−1, at a resolution of 4 cm−1.
X-ray diffraction (XRD) spectra were recorded using an X-ray diffractometer at 2𝜃 = 5–80°. XRD spectra were recorded using a Rigaku SmartLab X-ray diffractometer with a CuK𝛼 radiation (𝜆 = 1.5406 Å) in 2𝜃 range 5–80°. The crystallite sizes of the investigated samples were calculated from XRD data using Scherrer equation:
The a and c lattice parameters of the LDH hexagonal crystalline structure were calculated using d(110) and d(003) lattice spacing, respectively:
The specific surface area and porosity parameters were determined by a N2 adsorption–desorption technique at −196 °C with an Automated Gas Sorption Data Quantochrome Instrument. Specific surface area was calculated based on Brunauer–Emmet–Teller (BET) isotherm model, the pore volume was determined from isotherm at the end of capillary condensation and the pore size distribution from the desorption branch using Barret–Joyner–Halenda (BJH) method and Harkins–Jura standard isotherm. The pH measurement was performed with a pH meter (WTW Multi 9430-Inolab) equipped with a pH Sentix 98 sensor (±0.04 precision) with temperature compensation. The concentration of lead was determined by FAAS with a GBS Avanta spectrometer with air–acetylene flame and background correction with deuterium lamp. The concentration of Mg2+ was examined using inductively coupled plasma optical emission spectrometry (ICP-OES Avio 500 Perkin Elmer).
2.4. Sorption experiments of Pb(II) ions
The study of the sorption capacity of Pb(II) ions from aqueous solutions on P24(5), P24(15), P24(30), P1(30), P24(55) and P1(55) adsorbent materials was carried out in 100 mL polypropylene flasks, by adding 0.1 g of adsorbent in 50 mL of Pb(II) aqueous solution with initial concentration of 100 and 1500 mg/L, respectively. The pH of the initial solution was adjusted to 5.0 to prevent Pb(II) ion precipitation. The flasks were shaken on a mechanical shaker at a speed of 300 rpm for 2 h, at a temperature of 25 ± 2 °C. The concentration of Pb(II) was determined after sample filtration through a 0.45 μm nitrocellulose membrane and dilution, by FAAS. Prior to the metal ion adsorption studies, the kinetic studies were performed under the same conditions, for three different concentrations of lead (100, 500 and 1000 mg/L) at different times in the range of 5–120 min in order to study the influence of adsorption time on equilibrium sorption capacity (qe).
The removal percentage R% (Equation (1)) and the quantity of lead adsorbed qe (Equation (2)) were calculated using the following equation:
(1) |
(2) |
The effect of the solution pH was studied for all samples under the same conditions. A 200 mg⋅L−1 Pb(II) solution with initial pH values in the range 2–6, and temperature of 25 ± 2 °C was used. The experiments were performed in polypropylene flasks containing 10 mL of Pb(II) solution of each pH value and 0.02 g of sample. After stirring for 2 h, and subsequent filtration and dilution, the lead concentration in the solution was determined by FAAS. At the end of the sorption experiments, the solution pH was measured and compared to that of the initial solution.
3. Results and discussions
3.1. Characterization of LDH-based materials
All samples were structurally and texturally characterized before and after Pb(II) adsorption. In the last case, the samples were dried at 80 °C under vacuum prior the analysis.
The selected SEM images of the investigated samples (Figure 1) revealed their specific morphology, ranging from flower-like microspheres, branching from the center in all directions, to agglomerated structures in the form of distinct platelets. After adsorption, an increase in particle agglomeration was evident.
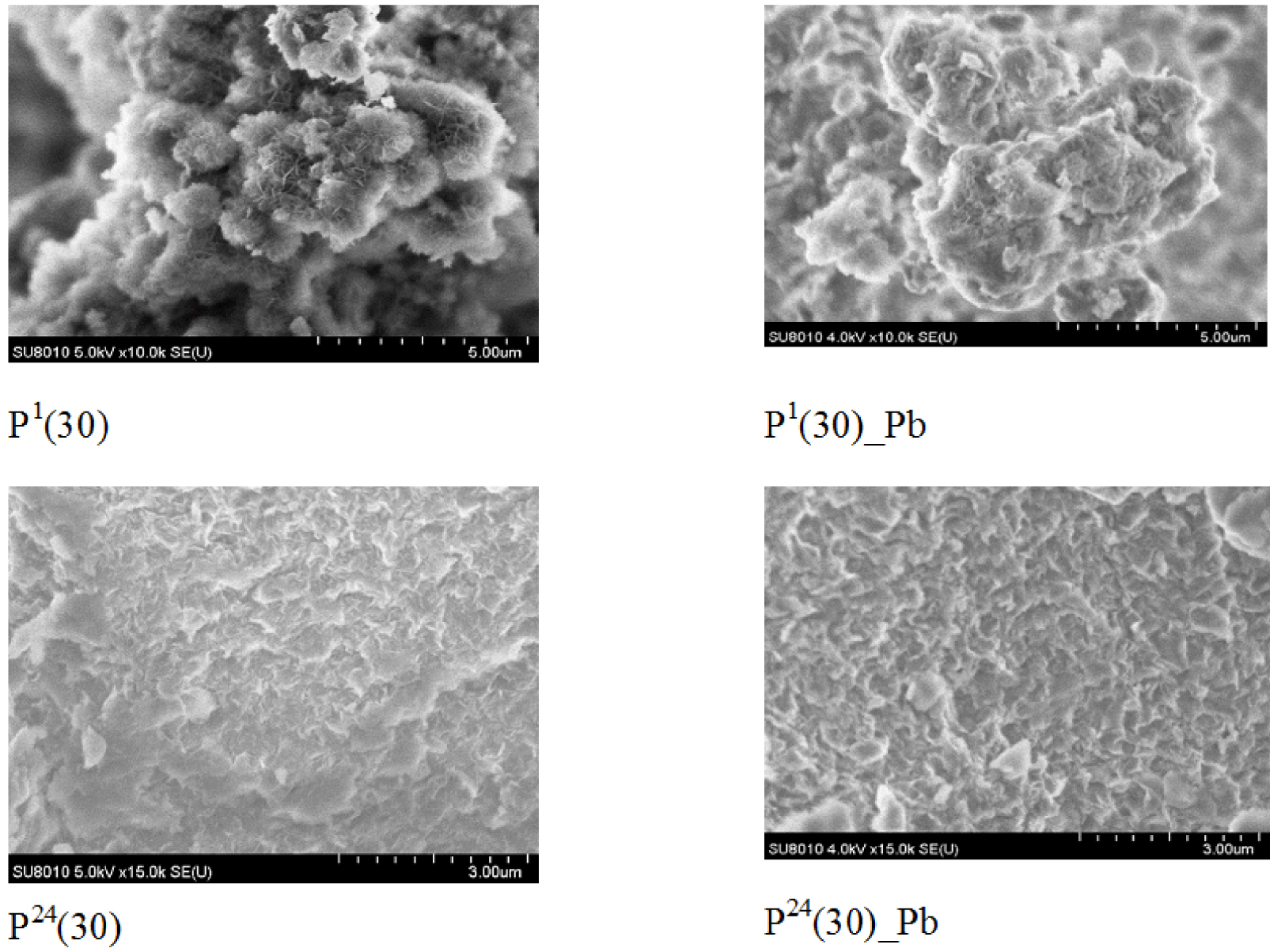
SEM images of LDH before and after metallic ion adsorption.
The elemental analysis of the samples from the EDX spectra (Table 1) indicated that P24(30) and P24(55) materials adsorbed the largest quantity of lead on their surface. When the MgAl–LDHs samples were immersed into the Pb(NO3)2 acidic solution, a part of Mg2+, HO− and
EDX analysis results of MgAl–LDH materials before and after Pb(II) adsorption
Atomic ratio | P24(5) | P24(15) | P24(30) | P1(30) | P24(55) | P1(55) | |||||||||||
---|---|---|---|---|---|---|---|---|---|---|---|---|---|---|---|---|---|
Before | After | Before | After | Before | After | Before | After | Before | After | Before | After | ||||||
Mg/Al | 2.00 | 1.93 | 1.51 | 1.65 | 2.20 | 2.33 | 2.71 | 2.71 | 2.40 | 2.49 | 2.39 | 2.93 | |||||
Pb/Al | - | 0.012 | - | 0.049 | - | 0.518 | - | 0.036 | - | 0.579 | - | 0.121 |
From the EDX analysis (Table 1) it was found that the observed Mg/Al ratios of the different analyzed samples did not correspond to the theoretical value (Mg/Al = 2). According to the results previously published [27], this is due to the pH fluctuations during the LDH synthesis. The minimization of the pH influence on Mg/Al ratio during LDH synthesis will be addressed in a future study.
The FTIR spectra of P1(30) and P24(30) samples are shown in Figure 2. Both spectra present broad maxima in the range 3700–3200 cm−1 ascribed to several contributions: the stretching vibrations of OH groups of the LDH layers and of water molecules between the brucite layers or on the material surface,

FTIR spectra of MgAl–LDHs obtained at 30 °C with different ageing times, before and after Pb(II) adsorption.
The peaks located in the range 770–600 cm−1 correspond to the lattice vibrations of the M–O, O–M–O, M–O–M bonds (M = Mg and Al) [31]. The intensity of the peaks in the 3500–3300 cm−1 region and in the carbonate/bicarbonate region increased after Pb(II) adsorption, indicating the formation of a higher quantity of Pb(OH)2, PbCO3 or Pb3(CO3)2(OH)2 during the adsorption process on the surface of LDH layers (Pb–LDH).
Figure 3 shows the XRD patterns of all MgAl–LDH samples before and after Pb(II) adsorption. All samples presented the characteristic peaks of LDH structure [32, 33], this being highlighted by the presence of high intensity peaks of the diffraction planes (003) (2𝜃 = 11.2°), (006) (2𝜃 = 22.8°), (009) (2𝜃 = 34.5°) and 2 peaks of low intensity corresponding to planes (110) and (113) (2𝜃 = 60–62°) [8]. The observed reflections can be indexed as the tree-layer 3R polytype with rhombohedral symmetry (space group
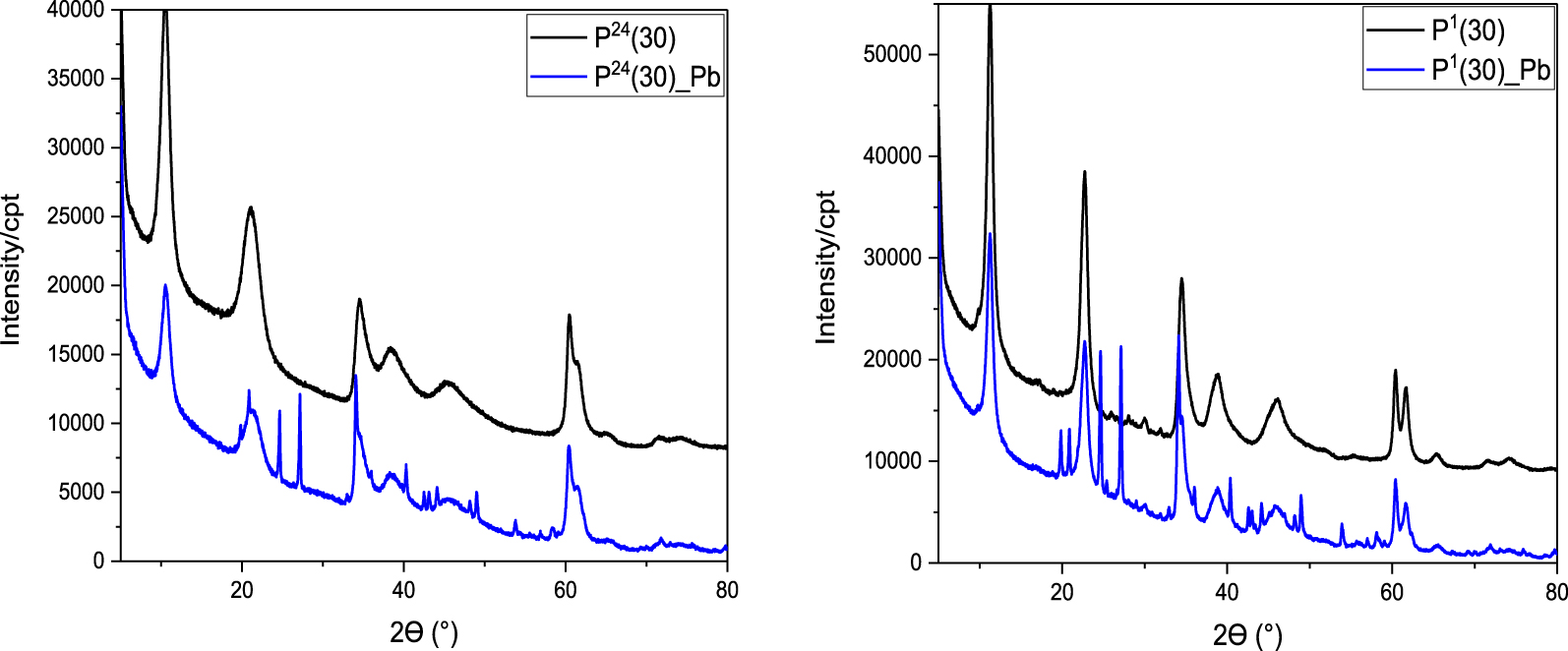
XRD patterns of MgAl–LDH materials obtained at 30 °C with different ageing times, before and after Pb(II) adsorption.
The basal spacing corresponding to the first reflection (003) (Table 2) is similar to hydrotalcite-type structure with carbonate in the interlayer (7.7 Å). It corresponds to the distance between the centers of two adjacents layers. Due to the presence of the interlayer anions, the LDH basal spacing is higher than the one corresponding to brucite, with a major influence of the anion size and the hydration extent. LDH interlayer galleries contain anions and water molecules, with a complex network of hydrogen bonds between hydroxyl groups, anions, and water molecules [33]. The lowest value was obtained for the sample prepared at 5 °C, while the samples synthesized at 30 °C exhibited the highest basal spacing independent of ageing time. The low basal spacing usually indicates a more tight packing of the ions and molecules in the interlayers [34]. In this context, an increase in the ageing temperature is expected to determine an increase in basal spacing due to a more lax packing of the constituent species in the interlayers.
Structural parameters of the investigated samples
Sample | d(003) (Å) | d(110) (Å) | c (Å) | a (Å) | D (nm) |
---|---|---|---|---|---|
P24(5) | 7.61(9) | 1.51(9) | 22.8(5) | 3.0(3) | 4.5 |
P24(5)_Pb | 7.72(1) | 1.51(2) | 23.1(6) | 3.0(3) | 7.9 |
P24(15) | 8.36(9) | 1.52(2) | 25.1(1) | 3.0(4) | 5.9 |
P24(15)_Pb | 8.24(9) | 1.52(1) | 24.7(4) | 3.0(4) | 6.0 |
P24(30) | 8.36(3) | 1.52(9) | 25.1(1) | 3.0(5) | 6.1 |
P24(30)_Pb | 8.36(9) | 1.53(2) | 25.1(1) | 3.0(6) | 7.6 |
P1(30) | 7.81(4) | 1.53(2) | 23.4(4) | 3.0(6) | 7.1 |
P1(30)_Pb | 7.81(1) | 1.52(9) | 23.4(4) | 3.0(6) | 7.7 |
P24(55) | 7.81(2) | 1.52(9) | 23.4(4) | 3.0(5) | 7.9 |
P24(55)_Pb | 7.72(1) | 1.52(7) | 23.1(6) | 3.0(5) | 8.5 |
P1(55) | 8.03(2) | 1.53(6) | 24.0(9) | 3.0(7) | 7.7 |
P1(55)_Pb | 7.81(4) | 1.53(1) | 23.4(4) | 3.0(6) | 8.9 |
In numerous studies addressed to MgAl–LDHs, the reported values of a lattice parameter were in the range 3.02–3.07 Å, lower than the one characteristic to brucite (3.142 Å). Moreover, they decrease with the increase in Mg/Al ratio. If there is no correlation between the a value and the apparent composition of LDH, this can be an indication of the presence of other non-LDH phases. There are several factors determinig the presence of broad asymmetric (01l)∕(10l) reflections in the XRD patterns of LDH-type powders, such as low crystallite sizes and the intergrowth of rhombohedral and hexagonal polytypes [33].
After the lead adsorption experiments, the characteristic diffraction lines of the lamellar structure of the LDH decrease in intensity and additional signals appear between 2𝜃 = 20–27° and 2𝜃 = 37–58°, which can be attributed to the corresponding crystalline phase Pb3(CO3)2(OH)2 according to JCPDS card no. 13-0131 [35]. Also, an increase in LDH-phase crystallite size was evident, the crystallite growth being promoted by the adsorption process (Table 2).
The textural properties of the MgAl–LDH-type adsorbents were determined from N2 adsorption–desorption isotherms at −196 °C. All the investigated samples exhibited type IV isotherms with a H2 hysteresis loop, characteristic of mesoporous LDH-type powders with small amount of micropores and aggregated plate-like particles [36].
The specific surface area, pore volume and average pore size are listed in Table 3.
Textural properties of MgAl–LDH adsorbents prepared in different synthesis conditions
Sample | BET surface area (m2∕g) | Pore volume (cm3∕g) | Pore size (Å) |
---|---|---|---|
P24(5) | 9.5 | 0.016 | 57.5 |
P24(15) | 19.0 | 0.104 | 79.6 |
P1(30) | 25.9 | 0.124 | 87.6 |
P24(30) | 36.3 | 0.236 | 93.0 |
P1(55) | 57.8 | 0.275 | 124.2 |
P24(55) | 73.2 | 0.337 | 174.4 |
If we compare the SBET of samples prepared at different maturation temperatures, the best results were obtained for samples synthesised at 55 °C. These samples exhibited the strongest agreement between the structural parameters (a and c from Table 2) with the characteristic ones of typical Mg/Al (2:1)–LDH [27]. All the textural parameters have increased when the maturation period increased from 1 h to 24 h, indicating an enhancement of the adsorption capacity.
3.2. Adsorption studies of Pb(II) pollutant
3.2.1. Sorption capacity of Pb(II) on MgAl–LDH adsorbents
Prior to the determination of sorption capacity of Pb(II) on MgAl–LDH adsorbents, the influence of contact time was studied for three different concentration of lead:100, 500 and 1000 mg/L, respectively. According to the adsorption profile for all concentrations (data not shown) the adsorption capacity of Pb(II) has increased rapidly in the first 20 min, and reaches an equilibrium with the quantity of adsorbed lead remaining constant after 2 h. For sorption studies, two Pb(II) ions concentrations were used, namely 100 mg/L, and 1500 mg/L, in order to determine the adsorption capacity of the tested materials. Figure 4 shows the sorption capacities of Pb(II) ions on the investigated samples and the corresponding removal percentage (R%). At an initial concentration of 100 mg/L, P24(55) sample absorbed the highest quantity of metal ion (47.25 mg/g) with a removal percentage of 94.5%. There are no significant differences either between samples synthesised at different temperatures and aged for 24 h or for the samples synthesised at different temperatures and aged for 1 h. At an initial concentration of 1500 mg/L, the sample synthesised at 55 °C and aged for 24 h exhibited the highest adsorption capacity, adsorbing 1151.97 mg/g with a corresponding removal percentage of 76.96%. The results revealed that at high metal concentrations, the synthesis temperature is the most important parameter for tailoring the material adsorption capacity, as this parameter increased with the increase in the synthesis temperature, while the effect of ageing period is smaller. When we consider both the structural and textural parameters in correlation with the Pb(II) adsorption capacity it is clear that the best adsorption capacity was obtained for the adsorbent possessing a crystalline structure as similar as possible to typical MgAl–LDH material (Table 2) and the best textural parameters (Table 3).
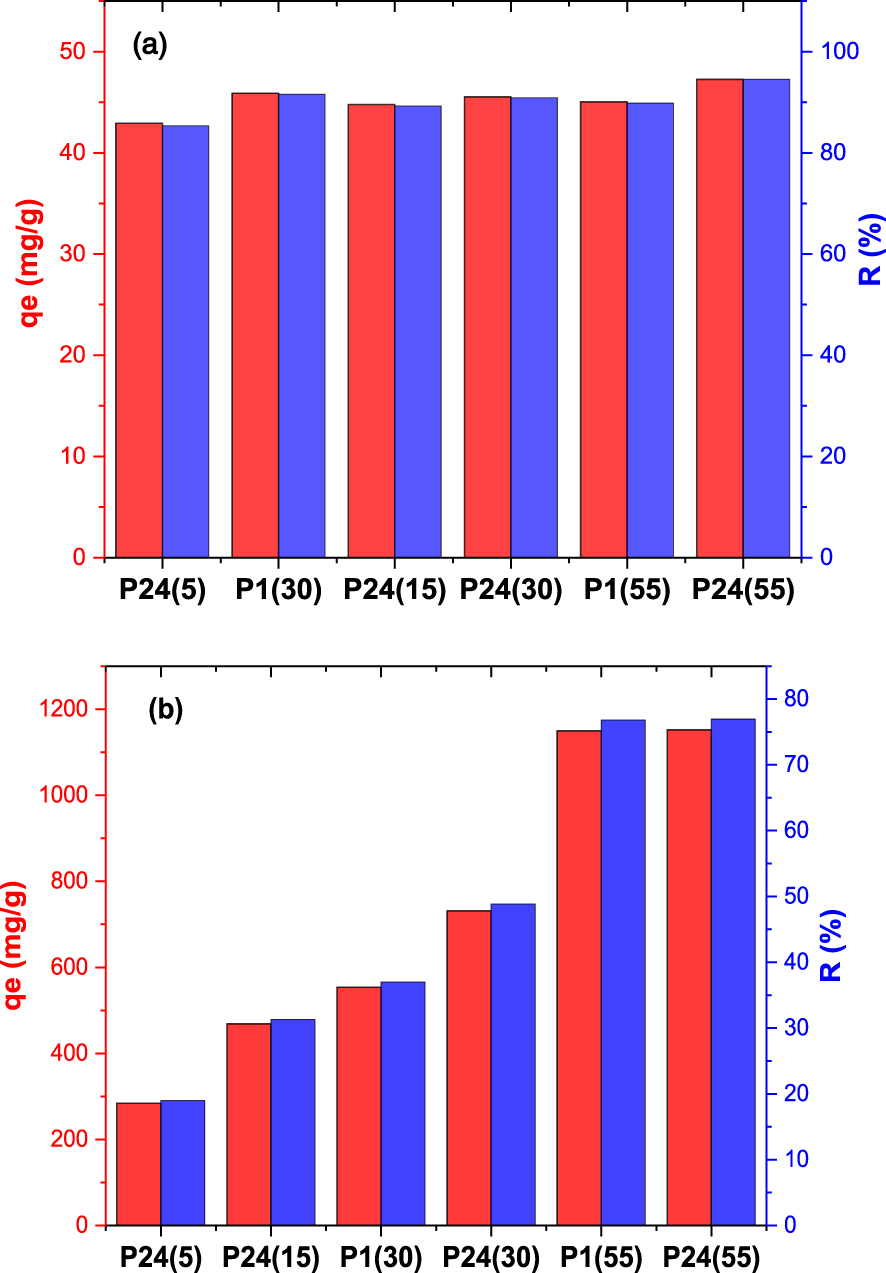
Adsorption capacity and the corresponding removal ratio (R%) for MgAl–LDH samples at (a) Ci = 100 mg/L and (b) Ci = 1500 mg/L.
Compared with the data from literature (Table 4), all the studied materials have similar or even higher adsorption capacities for Pb(II) ions, highlighting the potential of their use as adsorbent materials for heavy metal ions, both at low and high concentrations in the initial solution.
Pb(II) adsorption capacity on different adsorbent materials
Material | Pb(II) adsorption capacity (mg/g) | Ref. |
---|---|---|
FeMnMg–LDH | 421.42 | [13] |
Chitosan/MgAl–LDH nanocomposite | 333 | [34] |
ZnFe–LDH intercalated with citrate ions | 94.3 | [8] |
Fe3O4/GO/MgAl–LDH | 173 | [37 ] |
Polysulphide/MgAl–LDH | 483 | [38] |
Sulfonated lignin/MgAl–LDH | 123 | [39] |
MnO2/MgAl | 49.87 | [40 ] |
Glutamate/MgAl | 68.49 | [41] |
290 | [42 ] | |
Mg2Al–LDH | 66.16 | [19 ] |
MgFe–LDH | 865.36 | [20] |
MgAl–phenylalanine–LDH | 852 | [21] |
MgAl/LDH/carbon film modified by polydopamine | 1223.15 | [43] |
MgFeAl–CO3LDH | 117.86 | [44 ] |
FeMg–LDH@bentonite | 1397.62 | [45] |
MgAl layered double oxide (LDO) | 1336.80 | [46] |
Sludge biochar/ZnAl–LDH composite | 226.10 | [47] |
ZnAl–LDH intercalated with amino trimethylene phosphoric acid (ATMP) | 84.06 | [48] |
MgAl–LDH co-precipitation with different synthesis parameters | 1151.97 | This work |
3.2.2. Influence of pH on Pb(II) sorption on MgAl–LDH adsorbent materials
The effect of pH on adsorption of Pb ions is shown in Figure 5. To prevent Pb(II) precipitation at high pH values, adsorption experiments were performed at pH values lower than 6. At pH < 2 the dissolution of the adsorbent has to be considered, which can lead to a collapse of the lamellar structure. In general, for LDH materials very low pH values are avoided in adsorption studies [49]. Also, at low pH values a protonation of the active sites of the adsorbent takes place and thus an electrostatic repulsion occurs between these positive charged active sites and the positive metal ions, also positive.
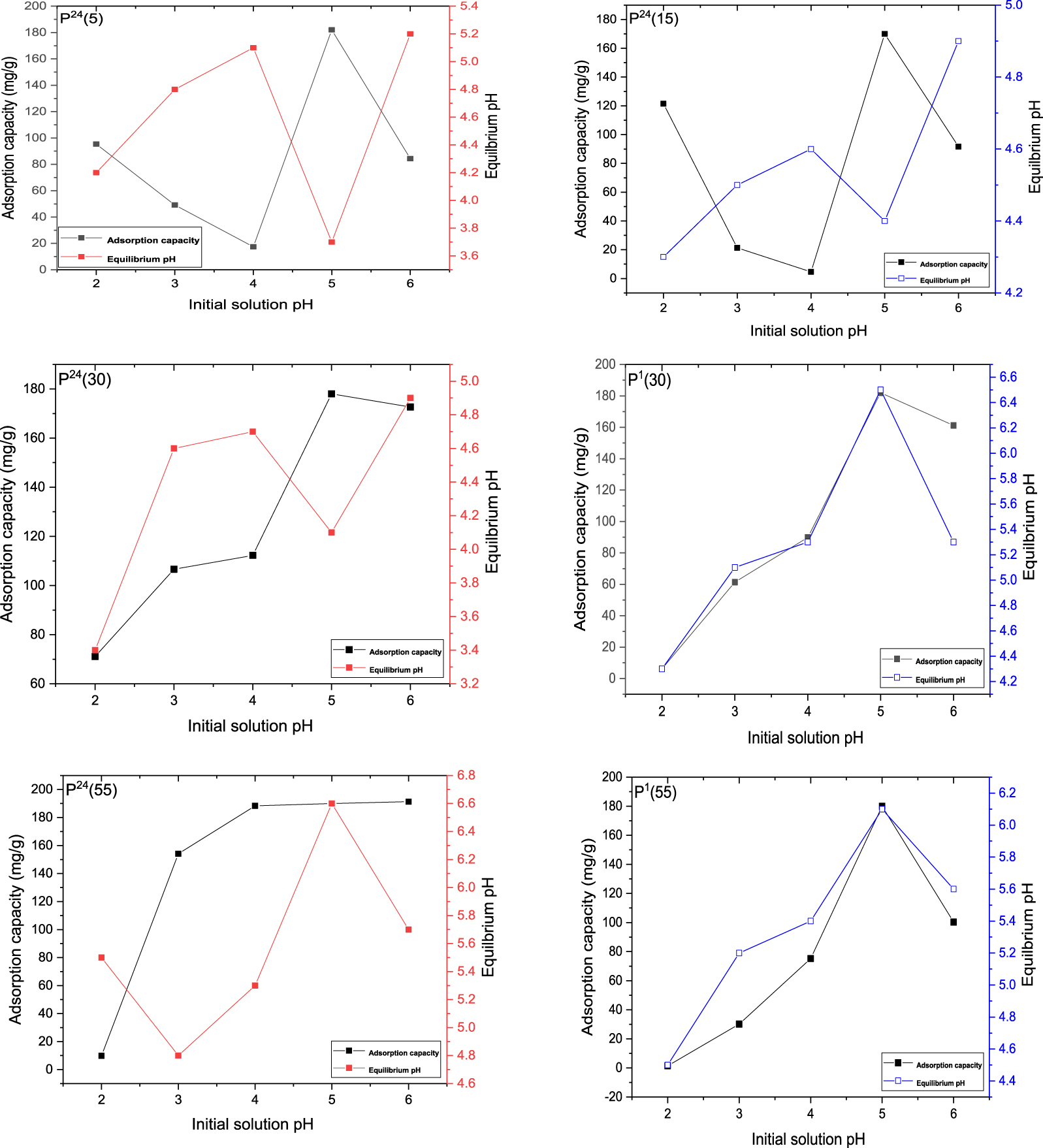
Effect of pH on the maximum absorption capacity of MgAl–LDH samples.
It was observed that the removal ratio of Pb(II) ions generally increases with pH, all materials exhibiting a maximum efficiency at a pH value of 5. P24(5) and P24(15) samples showed a decreased adsorption capacity when pH ranges between 2 and 4, followed by an increase in the maximum adsorption at pH = 5 and a subsequent decrease at pH = 6. The smaller adsorption capacity of lead in the pH range of 2–4, is probably due to a smaller quantity of hard-to-reach carbonate anions in their interlayer region, as the maturation temperature was not enough for carbonate retention in the interlamellar space. The same samples presented a higher adsorption capacity at pH = 2, due to an accelerated Mg leaching which favored the interlayer anions release. For P24(30), P1(30), P24(55) and P1(55) samples, an increase in Pb(II) adsorption was observed in the pH range 2–5, followed by a slight decrease (P24(30), P1(30), P1(55)), or a flattening (P24(55)) at the pH value of 6. As the pH increases from 2 to 5, electrostatic attractive forces become stronger and the surface of the LDH becomes more accessible for sorption of metal ions. The decrease in the removal ratio of Pb(II) ions at pH values greater than 5 can be explained by transformation of Pb(II) into Pb(OH)+, Pb(OH)2 or
Regarding the values of the final equilibrium pH, a different tendency was noticed. Thus, for samples P24(5), P24(15) and P24(30), the equilibrium pH values when an initial value of 2 was used, were 4.4, 4.2 and 3.4, respectively. When the initial pH is 2, the increase in final pH is probably due to the partial dissolution of the hydroxyl layers of LDHs; this behavior is also confirmed by the presence of Mg in the final solution. An increase in the final pH for the initial pH ranging between 2 and 4 was evident, up to the maximum values of 5.1 (P24(5)), 4.6 (P24(15)) and 4.7 (P24(30)), respectively. The pH changes could be associated with the continuous dissolution of the hydroxyl layers in order to produce the basic lead carbonate precipitation on the surface, as these pH values were not able to produce complete precipitation of Pb(II) [51]. Due to the different solubility products of lead carbonate (KSP = 1.5 × 10−13) and lead hydroxide (KSP = 2.8 × 10−16), the content of lead hydroxide probably exceeded that of carbonate [24]. At the initial pH of 5, where the adsorption capacity of Pb(II) was maximum, the equilibrium pH decreases to 3.6 (P24(5)), 4.4 (P24(15)) and 4.1 (P24(30)), respectively. This behavior can be explained by the existence of a complementary mechanism of complexation of Pb(II) with the –OH groups on the surface of LDHs at this pH, with the simultaneous release of the H+, which is predominant over precipitation [24]. This maximum adsorption pH value is under the point of zero charge (pHpzc) which was determined to be around 7.7 for this type of MgAl–LDHs [52]. Therefore, the surface of adsorbent is positively charged due to the protonation of surface –OH groups. However, the positive charge on the surface is relatively small at this pH, as the deprotonation of LDHs increases, in comparison with more acidic pH = 2, allowing an increase in lead complexation by surface –OH groups. For P1(30), P24(55) and P1(55) samples, there was an increase in the equilibrium pH with respect to its initial value in the range 2–5, with the maximum values in the range 4.4–6.6. In this case, the highest equilibrium pH values were recorded at the initial pH of 5, where the sorption capacity reaches a maximum. This behavior could be explained by a better buffering capacity of these materials compared to previous samples, due to the partial dissolution of the adsorbent and release of OH− to increase the pH. The increase in the equilibrium pH compared to the pH of the initial solution was also noticed for other LDH materials and was ascribed to their pH buffering capacity [13]. When an LDH–Cl hydrotalcite was used for the sorption of metal ions, the buffering capacity of the material led to a significant increase in pH [53], with precipitation of metal hydroxides being the main sorption mechanism. These hydroxides precipitated either as part of the LDH structure [51] or as separate phases [54].
For the investigated materials, it is likely that the sorption of Pb(II) ions occurs through co-precipitation mechanisms in the form of Pb(OH)2, PbCO3 or Pb3(CO3)2(OH)2-characteristic of LDH-type materials, but also through surface complexation by bonding with the surface hydroxyl groups. Correlating the studies of the pH influence with the concentrations of Mg determined by EDX and ICP-OES analysis, we can consider that for the P24(5), P24(15), P24(30) samples the surface complexation by the surface hydroxyl groups has a greater influence in the global mechanism of Pb(II) ions sorption, while for the P1(30), P24(55) and P1(55) samples, the precipitation mechanism is predominant. The increase in Mg concentration after Pb(II) sorption is due to a partial dissolution of LDHs in order to release the corresponding anions (OH− and
4. Conclusions
The purpose of this study was to obtain MgAl–LDH materials with improved properties toward the removal of Pb(II) ions by adjusting some of the synthesis parameters (temperature and ageing time).
The elemental analysis of the samples after adsorption indicated that P24(30) and P24(55) adsorbed the largest quantity of lead on the surface, with the preservation of their characteristic LDH lamellar structure. The stability of crystalline structure was also confirmed by XRD analysis for all the investigated samples. Moreover, XRD analysis revealed a decrease in the intensity of the characteristic peaks of the lamellar structure of the LDH after lead adsorption and the presence of additional peaks which can be attributed to the Pb3(CO3)2(OH)2 crystalline phase.
The lead adsorption experiments have shown that at an initial concentration of 100 mg/L, sample P24(55) absorbs the highest quantity of metal ion (47.25 mg/g) with a removal percentage of 94.5%. At the initial concentration of 1500 mg/L, the sample absorbed 1151.97 mg/g, with a removal percentage of 76.96%. The efficiency of Pb(II) ion removal generally increases with increasing pH value, all materials exhibiting a maximum efficiency at a pH value of 5.
Sorption of Pb(II) ions occurs through co-precipitation mechanisms in the form of Pb(OH)2, PbCO3 or Pb3(CO3)2(OH)2 characteristic to LDH materials, but also through surface complexation by bonding with the surface hydroxyl groups. By correlating the results of pH influence with the Mg concentrations determined by EDX and ICP-OES analysis we can conclude that for the P24(5), P24(15), P24(30) samples, the surface complexation by the surface hydroxyl groups has a greater influence in the global mechanism of Pb(II) ion sorption, while for the P24(30), P1(30), P24(55) and P1(55) samples, the increase in Mg concentration after Pb(II) sorption was due to their higher pH buffering capacity made by partial dissolution of the adsorbent and release of OH− to increase the pH.
Further thermodynamic and kinetic studies will be performed on these materials in order to develop an efficient procedure for wastewater purification.
Conflicts of interest
Authors have no conflict of interest to declare.