1 Introduction
For centuries, the search for new materials with precise characteristics and applications has been one of the most important stimulants of technological development [1]. In general, to fabricate the most simple or complex device or object, it is essential to search for materials that exhibit the desired physico-chemical properties for the aspired application. But can a particular material, with identical chemical composition and structural arrangement, display different properties? The answer is yes, but this largely depends on the dimensions of the material itself or the dimensions of the components [2,3].
Outstanding properties can arise when materials are in the nanometric scale, but this is not only due to dimensional features, like the surface/volume ratio (very high for nanostructures), but it also depends on the form, environment, and organisation of the particles that comprise the nanostructured material. Indeed, these features are consequence of a “nano-effect”, and most of them are associated with quantum-like laws [4,5].
Metal particles, like Au, Ag, and Cu, are known to absorb specific wavelengths of the visible spectrum when they possess nanometric dimensions. This singular absorption is due to the collective resonant oscillation of the electrons of the conducting band promoted by the electric field of the incident light at the particle surface [6,7]. This effect is called surface plasmon resonance (SPR), and was first elucidated by Mie in 1908 [8,9]. For that reason, for example, the optical properties of gold nanoparticles (AuNPs) can be significantly modified if their dimensions are in the range of 1 to 100 nm [10,11]. Indeed, with precise synthesis control, it is possible to prepare colloidal solutions of AuNPs with different colours [12].
Spherical AuNPs typically display one absorption band in the visible or near-infrared spectrum, and just one range of these electromagnetic radiations can induce the expected SPR (Fig. 1). On the other hand, anisotropic nanoparticles display different absorption bands since different SPR energies are generated [6]. Nanorods and nanowires, for example, display two typical absorption bands; one related to the longitudinal, and the other to the transversal SPR (Fig. 1) [13].
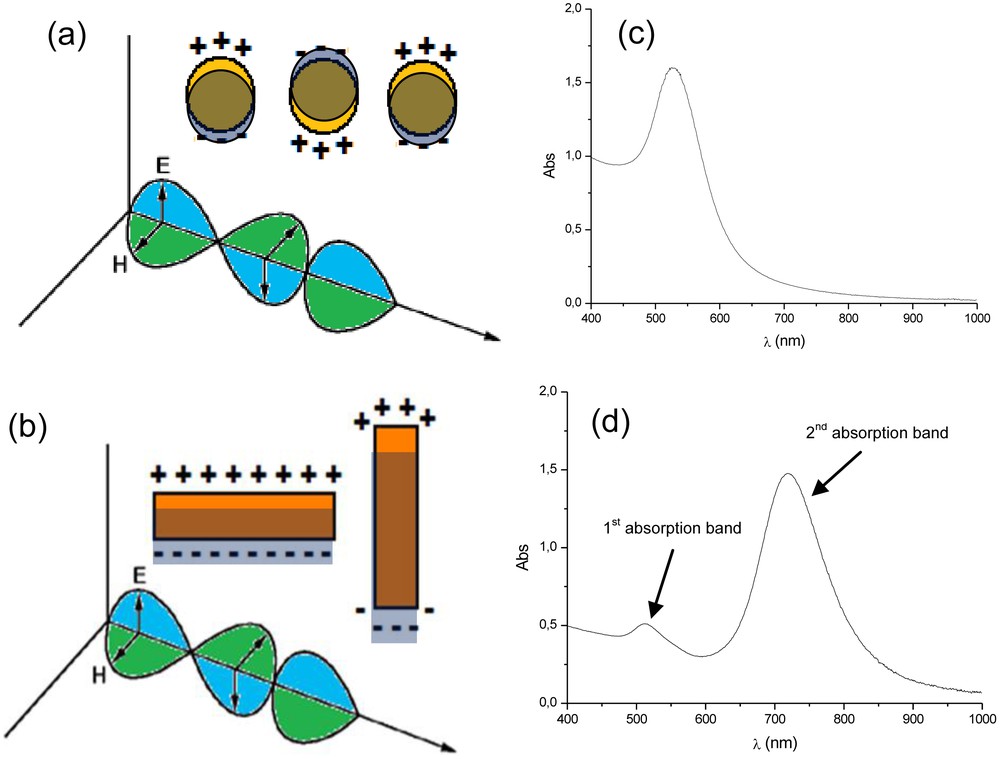
(a) Illustration showing the single surface plasmon resonance on a spherical (a) and rod-like (b) gold nanoparticles, generated due to the interaction of the particle with the electromagnetic field of the incident light. (c) and (d) are an illustration of the respective typical outline UV–vis absorption spectrum expected for those colloidal solutions.
It is worth noting that one can profit from the optical features of AuNP-based structured systems and use relatively simple UV–vis(-NIR) spectroscopy to evaluate and follow the synthetic procedures and the characteristics of the final product [14,15].
This great versatility of colloidal systems of AuNPs, in terms of optical properties, continues to attract the attention of several research groups. New synthetic strategies to obtain specific AuNPs in high yields, for precise applications [16,17], are frequently observed in the modern literature [10,18,19]. In addition to studies in the field of photonics [20] and optics [6,21], applications of AuNPs can also be found in areas like catalysis [22], sensors [23], medical diagnosis [24,25], and tumour treatment [26,27].
Metal nanoparticles can be prepared on the bases of physical and chemical methods, following two main approaches: (i) bottom-up or (ii) top-down methodologies. Nanoparticles obtained via the top-down approach are based on physical methods, and are normally generated from larger particles. Sputtering [18,28,29], sonolysis [30], and nanolithography [31,32] are examples of techniques based on this practice. On the other hand, methods based on “wet chemistry” follow, in general, the bottom-up approach. In this latter case, nanoparticles are frequently prepared from molecular metal precursors that, after specific chemical transformations, generate very reactive “solvated” metal atoms that soon interact to each other via metal–metal bonds until they reach the size and shape of the desired metal nanoparticle. These nanoparticles remain, in many cases, dispersed in the liquid matrix, like colloidal solutions [33,34].
Of all the possible metal nanoparticle shapes that can be generated, the spherical ones are the most thermodynamically stable, since, with this shape, the lowest surface potential is achieved, and, for this reason, the particles are easier to obtain. However, depending on the concentration of the metal source in the solution and/or the presence of particular chemical compounds (growth-driving agents), other forms of nanoparticles can be obtained [35,36]. Indeed, there are a series of studies that report on the preparation of nanoparticles of several different forms, i.e. tetrahedral [37], cubic [38], prismatic [39], cylindrical [40], star-like [41], hollow [42], disk [43], etc. [44].
Several research groups have focused on anisotropic nanoparticle synthesis, especially because of their optical properties, which are closely related to the presence of different surface plasmons [45]. The most common strategies used to control the shape and size of anisotropic nanoparticles are based on wet chemistry methodologies [46–50].
Among the range of shapes possible for AuNP, rod-like particles are particularly well studied. In particular, many aspects of AuNRs synthesis have been discussed in the literature, especially regarding how to obtain particles with different sizes and shapes [2,44,51,52]. Indeed, AuNRs can be obtained with a high level of morphological control and different aspect ratios (the ratio between the particle length and width). They can also achieve dimensions of a few nanometres to ca. 1 μm [16,44,53–55].
Different procedures can be used to obtain AuNRs [56–59], but the seed-mediated methods are the most commonly employed [60]. The reference works by Murphy et al. [44,61] and El-Sayed et al. [62] showed that AuNRs can be prepared from very small AuNPs (“seeds” of 3 to 4 nm in diameter), which act as nucleation sites for the formation of the desired particles. The seeds are added into a growth solution, which basically contain AuI species that can be seen as the “monomer precursors” that are easily reduced to Au(0) at the surface of an AuNP in the presence of a mild reducing agent, such as ascorbic acid (AA). A small amount of silver nitrate (AgNO3) is also added to the growth solution. It seems to play an important role in obtaining AuNRs in high yields, but its role still incites discussion [63].
It is important to remark that, in this methodology, the presence of a chemical species that interact with the growing particle is essential, as it acts as a growth-driving agent – normally surfactants, like cetyltrimethylammonium bromide (CTAB). The role of these molecules is not just for particle protection, by avoiding particle agglomeration, but also to allow dissymmetric particle growth [47,64–68].
In this case, dissymmetric growth is mainly due to the dissimilar force interactions between the distinct crystal facets of the growing particle, which display different surface potentials [69], and the growth-driving agent. This leads to distinct rates of facet growth, permitting the formation of anisotropic nanoparticles. In other words, some facets are blocked by the growth-driving agent, provoking different rates of facet growth [64].
Fig. 2 illustrates, in a very simple way, the dissymmetrical growth mechanism of AuNRs. Of course, other factors like reaction temperature [70], seed and/or metal source concentrations [60], and surfactant chemical structure and concentration are variables that must be controlled for optimal AuNR production [67,71].
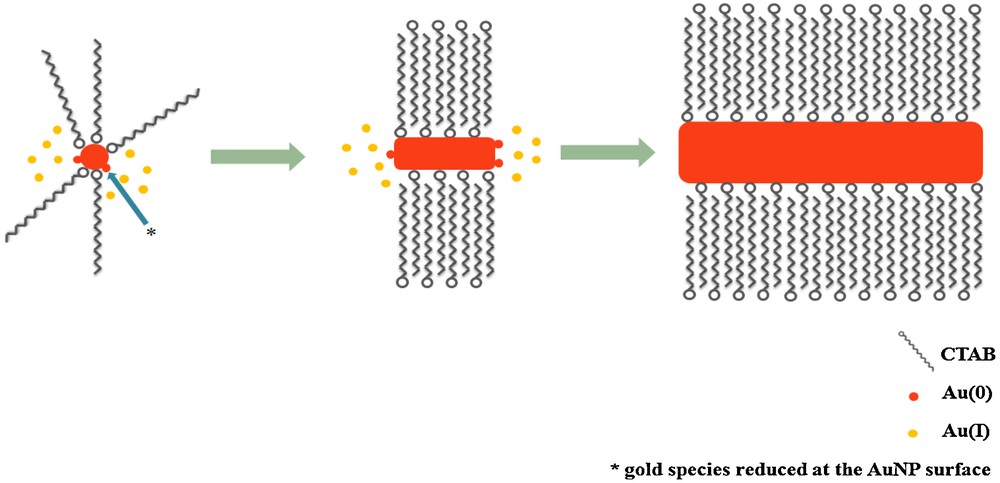
Schematic illustration of the dissymmetric growth of an gold nanorods from a seed particle and AuI ion species that are reduced to Au(0) at the surface of the metal particle.
Although AuNRs can currently be produced with a high level of control, with different sizes, aspect ratios, and low polydispersity, there are some aspects of their formation that are not yet fully understood. It is in this context that this study was proposed, to improve our understanding of AuNR formation.
2 Experimental
2.1 Materials
Tetrachloroauric acid (HAuCl4·3 H2O, 99.9%, Aldrich), sodium borohydride (NaBH4, > 98%, Acros Organics), AA (99%, Vetec), CTAB (99%, Acros Organics) and AgNO3 (> 99%, Reagen) were used as purchased. Deionised water (Deionizer Direct–Q3 Millipore) was used in the preparation of all aqueous solutions. All the glassware was cleaned with aqua regia and rinsed with deionised water prior to experiments.
2.2 Synthesis of gold nanorods
All colloidal solutions containing AuNR were prepared from the mixture of two solutions, i.e. the seed and growth solutions, according to seed-mediated methods, and adapted from reference [54].
The seed solution was prepared in a 20 mL flask in which two aqueous solutions of HAuCl4·3 H2O (5.0 mL; 0.5 mmol/L) and CTAB (2.5 mL; 0.20 mol/L) were added. In the sequence, an ice-cold aqueous solution of NaBH4 (0.6 mL; 0.01 mol/L) was added at one time. Immediately, the colour changed from dark yellow to brownish. The resulting solution was kept under gentle magnetic stirring for 2 min. The resulting solution was used for a period of 2 h after the preparation. This solution contained ca. 1.25 × 1014 particles of 4 nm/mL, and was used in all experiments.
According to the experiment, different growth solutions were prepared. For example, for illustration, in a 20 mL glass tube, three aqueous solutions of HAuCl4·3 H2O (5.0 mL; 1.0 mmol/L), CTAB (2.5 mL; 0.20 mol/L), and AgNO3 (0.150 mL; 0.40 mmol/L) were added. Under stirring, an aqueous solution of AA (0.070 mL; 0.80 mmol/L) was added all at once. Immediately, the colour changed from yellow to colourless.
Finally, the different colloids containing AuNRs were obtained by mixing appropriate quantities of seed and growth solutions, as well as CTAB. In a typical experiment, the seed solution (12 μL) was added, under gentle stirring for 10 s, into a recently prepared growth solution (7.72 mL; 0.64 mmol/L of AuI species), and left undisturbed for at least 4 h.
2.3 Characterisation
Electronic absorption spectra of the colloidal solutions were obtained using a Varian Model Cary 50 Scan UV-Vis/near-IR spectrophotometer. The setup was configured to fix the baseline of the deionised water absorption band from 400 to 1 000 nm, using quartz cells with path length of 1.0 cm. The particle size and shape analysis of the AuNRs were performed using a FEI-Tecnai 20 transmission electron microscope operating at 200 kV or a FEI–Morgani 268D operating at 100 kV. The samples were prepared by evaporating a thin film of nanoparticle solution on Holey carbon or formvar-coated copper grids for at least 24 h in a desiccator. Micrographs from transmission electron microscopy (TEM) were evaluated using the Sigma Scan program to determine the particle size distribution, measuring approximately 100 nanoparticles from each sample.
3 Results and discussion
The AuNRs were prepared by the seed-mediated method, adapted from the method developed by Murphy et al. [71]. As already mentioned, this method leads to high yields of AuNRs with a high degree of size and aspect ratio control. Nevertheless, slight changes in the synthesis conditions, for example, the quality of the water used to prepare the solutions, the amount of matter, and the concentration of the components, can induce significant modifications on the features of the AuNR. For this reason, we carried out a systematic study related to the formation of AuNR by modifying three parameters of synthesis, i.e. CTAB, seed, and monomer amounts in the reaction medium. It is worth remarking that all analyses performed here used samples on which no previous treatment has been carried out.
3.1 Kinetic study of gold nanorods formation
In order to verify the dynamic of formation and range of stability of the aqueous AuNR-based colloids, we carried out a kinetic study of particle formation using UV–vis spectroscopy; (Fig. 3). We evaluated the growth process (AuNR formation) from the first moments until a period of one week, taking a series of electronic absorption spectra of samples prepared like solution B (Table 1). During this time, the colloid displayed dynamic behaviour in terms of colour change and colour intensity. Nevertheless, it is possible to divide the formation process of AuNRs into three main stages after the addition of the seed into the growth solution:
- • the period from 0 to 2 h is characterised by the time needed for the AuNRs to form, with depletion of the AuI source;
- • from 2 to 48 h, no significant change in the colloidal system is detected. This is the best period in which the colloid can be manipulated and studied;
- • from two days on, the main mechanism of the growth process seems to be characterised by the occurrence of Ostwald ripening [72], i.e. larger particles continue to grow, while smaller ones (more unstable, with a higher surface potential) get smaller [2].

Absorption spectra of the colloid containing the gold nanorods recorded from the first moments of nanorod formation until day 6 (Top). Graphics (a) and (b) indicate the evolution of the maximum absorption intensity of the second band as a function of time, during the first 6 h and 7 days, respectively. Graphics (c) and (d) indicate the evolution of the of maximum absorption wavelength of the second band as a function of time.
Reaction conditionsa for the preparation of gold nanorods in the presence of different amounts of CTAB and some characteristics of the colloids obtained.
Sample | Volume of CTAB stock solution (mL)b | [CTAB] (mmol/L)c | CTAB:Au molar ratio | λmax1 (nm) | λmax2 (nm) | AuNR length (nm) | Aspect ratio |
A | 5.0 | 97 | 200 | 514 | 730 | 45 ± 4 | 2.8 |
B | 2.5 | 64 | 100 | 515 | 732 | 45 ± 3 | 3.0 |
C | 1.0 | 32 | 40 | 513 | 735 | 50 ± 10 | – |
Dd | 0.5 | 17 | 20 | 527 | 775 | – | – |
a Reagent amounts employed: 5.0 mL of tetrachloroauric acid (HAuCl4·3 H2O) (1.0 mmol/), 0.150 mL of silver nitrate (AgNO3) (4.0 mmol/), 0.070 mL of ascorbic acid (80 mmol/), and 12 μL of seed solution.
b Concentration of the CTAB stock solution: 0.20 mmol/L.
c Concentration of the CTAB in the colloidal system.
d Low yield of AuNR, large amount of spherical AuNP are present.
This last process explains the significant increase in the absorption bands. In this case, the smaller particles are a second reservoir of gold species for the growth process.
In the first hour of particle formation, it was possible to verify the regular growth of nanorods since the intensities of their respective surface plasmon absortion bands increased relatively quickly. After this stage, the intesities of the absorption bands did not change significantly over a period of 48 h. However, from this period on, Ostwald ripening dictated the growth process, characterised by an enhancement in the intensity of the plasmon absorption bands (Fig. 3).
It was also interesting to note that, during the first 2 h of AuNR formation, the maximum absorption of the second band shifted to the red region of the UV–vis spectrum and turned back (blue shift) to around 790 nm (maintaining this wavelength and absorption intensity from 4 to 48 h after the start of the particle formation; Fig. 3). The observation of this shift suggests that there is a significant change in the aspect ratio (length × width) of the particles during particle formation.
3.2 Effect of the CTAB:Au molar ratio
In this study, four growth solutions containing relatively low and high CTAB:Au molar ratios were prepared. Those solutions contained different amounts of CTAB, keeping the other component quantities constant. Into them, the same amount of seed particles was added. According to the method employed, the same amount of particles must be formed in all solutions, since the same amounts of seeds were used. The reaction conditions for this study are summarised in Table 1.
Fig. 4 shows the electronic absorption spectra of the colloids obtained 24 h after the addition of the seeds in the respective growth solution. In all measurements, the presence of two maximum absorption bands that are typical for colloids containing AuNRs was observed.The resulting spectra also show different correlations between the two absorptions bands (λmax1 < λmax2), which can be an indication of AuNR formation with different aspect ratios. Additionally, one can observe that the relative intensity between the two maximum absorptions in each spectrum are different. According to the optical law, the extinction coefficient of a metal nanoparticle is dependent on its size, and the longitudinal section has a higher extinction coefficient than the transversal one [9]. Thus, a typical UV–vis absorption spectrum of a colloid containing high numbers of AuNRs must have its λmax1 less intensive than its correspondent λmax2. This was the case for samples A and B, suggesting that we attained AuNRs in high yields. This was not the case for samples C and D, in which other forms of nanoparticles were present, probably the more thermodynamically stable spherical ones.
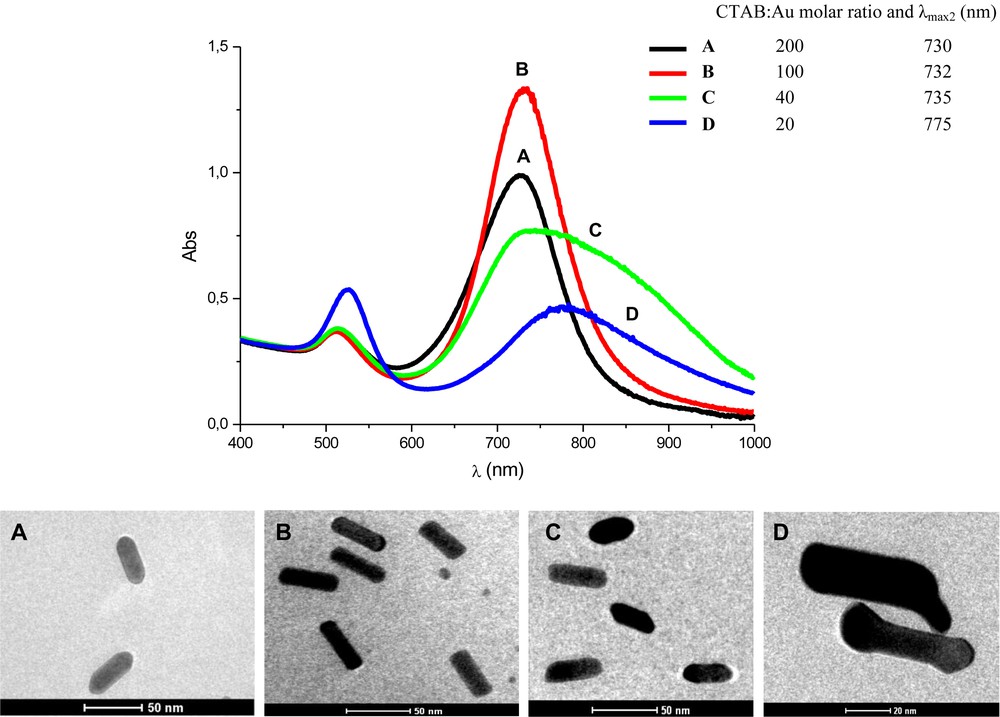
Electronic absorption spectra of the colloids with different CTAB:Au molar ratio, obtained 24 h after rods formation. Sample A, B, C and D prepared with CTAB:Au molar ratio of 200, 100, 40, and 20, respectively (Top). TEM images showing the typical size and shape of the respective AuNRs obtained.
The conclusions derived from the UV–vis absorption spectra were corroborated by the TEM images (Fig. 4). Most of the nanoparticles observed were rod-like and very homogenous in samples A and B. Both samples presented the same aspect ratio (3.0), with length and width around 45 and 15 nm, respectively (a slightly higher degree of homogeneity was seen in sample B). As we decreased the CTAB:Au molar ratio, as in the solutions C and D, the rod-shape appearance was strongly affected, leading to irregular rod-shaped particles and other different irregular forms.
Indeed, in this study, it was possible to verify that if we carried out a synthesis with CTAB:Au molar ratios between 100 and 200, we could reach a safe range of work to obtain AuNRs with the same size and aspect ratio, without significant variations, i.e. AuNRs with a length of 45 nm and an aspect ratio of 3.0.
3.3 Effect of the number of seeds
AuNRs were prepared with the addition of different numbers of seed particles into identical growth solutions (7.72 mL; CTAB:Au molar ratio of 100; 0.64 mmol/L of AuI). The respective experimental conditions adopted in this study are presented in Table 2, as well as some of the respective experimental results. Fig. 5 shows the UV–vis absorption spectra and TEM images of the particles obtained after 24 h of AuNR formation.
Reaction conditionsa for the preparation of gold nanorods (AuNRs) in the presence of different amounts of seed particles and characteristics of the particles obtained.
Sample | Seed solutionb (μL) | AuI:seedc x 105 | λmax1 (nm) | λmax2 (nm) | AuNR length (nm) | Aspect ratio |
E | 2.4 | 100 | 516 | 650 | 56 ± 3 | 1.7 |
B | 12 | 20 | 511 | 732 | 45 ± 3 | 3.0 |
F | 60 | 4.0 | 509 | 784 | 42 ± 4 | 3.7 |
G | 120 | 2.0 | 509 | 777 | 54 ± 4 | 3.6 |
Hc | 300 | 0.8 | 511 | 770 | – | – |
Ic | 600 | 0.4 | 516 | 707 | – | – |
a The growth solution was prepared from: 5.0 mL of AuIII (1.0 mmol/L), 2.5 mL of CTAB (0.2 mol/L), 0.150 mL of AgNO3 (4.0 mmol/L), and 0.070 mL of AA (80 mmol/L).
b Ratio of AuI species and seed particles in the reaction medium.
c Dog-bone shape AuNRs predominate.
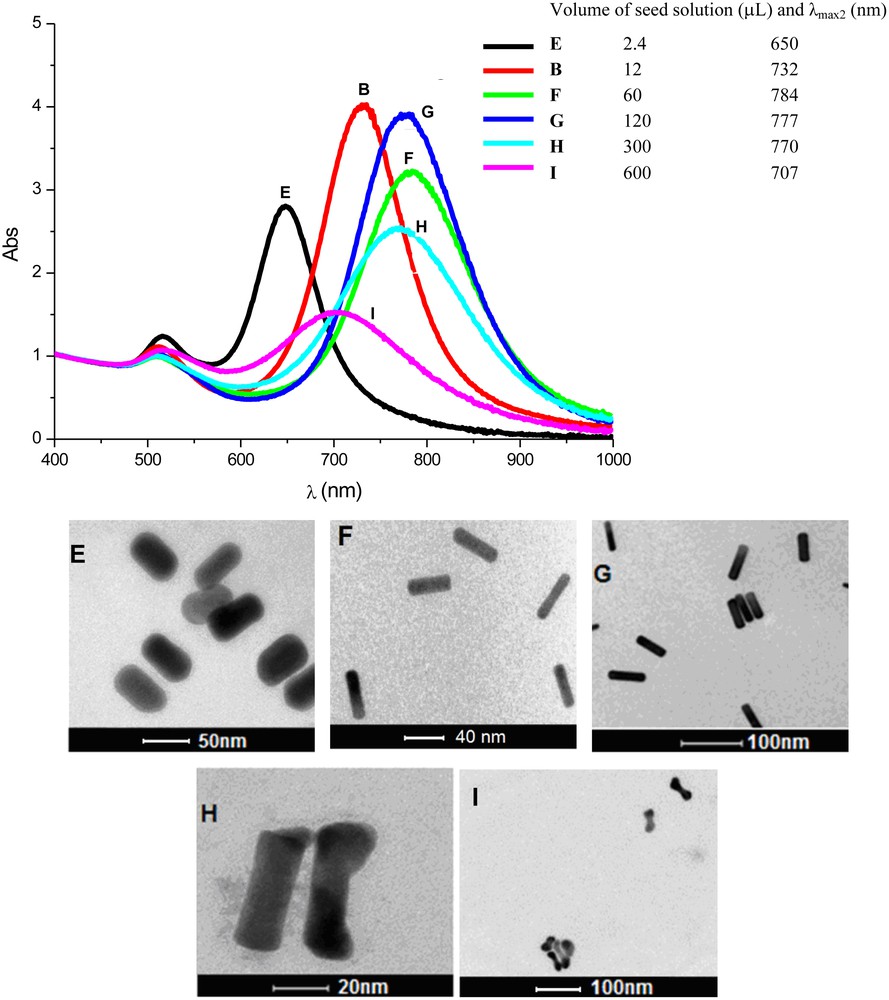
Absorption spectra of the colloids containing gold nanorods prepared with different amounts of seed particles in the reaction medium, after 24 h. Samples B, E, F, G, H, and I were prepared with different amonts of seed particles (top). TEM images of the respective gold nanorods prepared from different amounts of seed particles. (E) 2.4 μL; (B) 12 μL; (F) 60 μL; (G) 120 μL; (H) 300 μL; (I) 600 μL added to the growth solution.
At this point, it is important to consider that the number of AuNRs formed is the same as the number of seed particles added. Thus, if we increase the amount of seeds added, keeping constant the amount of AuI species in the solution, i.e. if the AuI:seed ratio decreases, smaller particles are produced.
From the data depicted on Table 2 and Fig. 5, one can see that solution E (2.4 μL of seed solution added) led to larger AuNRs; however, their aspect ratios were smaller (∼2.0). Indeed, we can see that, until a certain level, by increasing the number of seeds in the medium (B < F < G), the aspect ratio of the AuNR also increased. If one were to increase even more the number of seed particles in the growth solution, the amount of AuI species (“monomers”) becomes too scarce to produce rod-shape particles with elevated aspect ratios. Furthermore, by adding more seeds to the growth solution, more AuNRs are formed, but of a smaller size; consequently, the CTAB necessary to produce the double layer around the particles becomes scarce. This also explains the production of dog-bone-shaped particles, since, at a certain level of rod growth, the amount of CTAB becomes insufficient to drive the preferential access of “monomers” at the tip of the rods during formation [19,73,74]. This study confirms that there is an ideal relation between “monomers” and seeds in the reaction medium to achieve highly selective production of rod-like particles, as verified in solutions and G [71].
3.4 Effect of monomer amount
In this study, we carried out two different set of experiments in order to evaluate the influence of the “monomer” (AuI) amount on the production of AuNRs. In both studies, we added the same amount of seeds into growth solutions with different amounts of “monomers”.
3.4.1 Method 1
In this first set of experiments, we added the same amount of seed solution (120 μL) into different volumes of growth solutions with the same characteristics (0.64 mmol/L of AuI species; CTAB:Au molar ratio of 100). Table 3 summarises the experimental conditions adopted and shows some experimental results. Fig. 6 shows the UV–vis absorption spectra and TEM images of the particles obtained 24 h after AuNR formation started.
Reaction conditionsa for the preparation of gold nanorods in the presence of different volumes of growth solution (0.64 mmol/L of AuI species) and characteristics of the particles obtained.
Sample | V (mL) of AuIII (1.0 mmol/L) | V (mL) of CTAB (0.2 mol/L) | V (mL) of AgNO3 (4.0 mmol/L) | V of AA (mL) | Total V (mL) | AuI:seedsb x 105 | λmax1 (nm) | λmax2 (nm) | AuNR length (nm) | Aspect ratio |
Jc | 2.50 | 1.25 | 0.075 | 0.035 | 3.86 | 1.0 | 509 | 697 | 22 ± 3 | 3.2 |
Gd | 5.00 | 2.50 | 0.150 | 0.070 | 7.72 | 2.0 | 509 | 777 | 54 ± 4 | 3.6 |
K | 10.0 | 5.00 | 0.300 | 0.140 | 15.4 | 4.0 | 510 | 774 | 38 ± 6 | 4.0 |
L | 20.0 | 10.0 | 0.600 | 0.280 | 30.9 | 8.0 | 513 | 725 | 32 ± 5 | 2.9 |
M | 50.0 | 25.0 | 1.50 | 0.700 | 77.2 | 20 | 514 | 720 | 11 ± 4 | 2.2 |
a Seed solution: 120 μL.
b Ratio of AuI species and seed particles in the reaction medium.
c Many spherical nanoparticles also present.
d TEM images, (Fig. 5).

Absorption spectra of the colloids containing gold nanorods prepared with different amounts of “monomers” in the reaction medium. All spectra were obtained 24 h after rod formation. Samples J, G, K, L, and M were prepared from different volumes of the growth solution (top). TEM images of gold nanorods prepared from different volumes of the growth solution (0.64 mmol/L of AuI species): (J) 2.5 mL;(K) 10 mL;(L); 20 mL;(M) 50 mL. Volume of seed solution added: 120 μL.
Using the reaction conditions adopted here, one can see that AuNRs were produced in all tests, but with different aspect ratios. Again, it was possible to verify that there is an ideal ratio between the amount of “monomers” and seeds in the reaction medium to obtain rod nanoparticles with higher aspect ratios. As expected, it is possible to observe that as we increased the volume of the growth solution, larger particles were obtained, but this does not mean that the aspect ratio increased. This trend was also observed in the previous study (compare with Fig. 5).
3.4.2 Method 2
A second set of experiments was performed in order to keep the same amount of species as in the previous test; however, the final volume of the colloidal solution of AuNRs, in all cases, was the same (∼8.0 mL). Table 4 summarises the reaction conditions adopted in this study. Fig. 7 shows the UV–vis absorption spectra and TEM images of the particles obtained 24 h after AuNR formation started.
Reaction conditionsa for the preparation of gold nanorods in the presence of different amounts of reagents, but with the same final volume of the colloidal solution of gold nanorods (∼8.0 mL), and characteristics of the particles obtained.
Sample | [AuIII] (mmol/L) | [CTAB] (mmol/L) | [AgI] (mmol/L) | [AA] (mmol/L) | AuI:seedsb × 105 | λmax1 (nm) | λmax2 (nm) | AuNR length (nm) | Aspect ratio |
Nc | 0.32 | 32 | 0.20 | 0.40 | 1.0 | 518 | 725 | – | – |
Gd | 0.64 | 64 | 0.40 | 0.80 | 2.0 | 509 | 777 | 54 ± 4 | 3.6 |
O | 1.28 | 128 | 0.80 | 1.6 | 4.0 | 512 | 734 | 50 ± 4 | 3.1 |
P | 2.56 | 256 | 1.6 | 2.4 | 8.0 | 518 | 645 | 4 ± 5 | 1.8 |
Qe | 6.40 | 640 | 4.0 | 8.0 | 20 | – | – | – | – |
a The final volume of the colloidal systems were ca. 8.0 mL; seed solution (120 μL); CTAB:Au molar ratio = 100.
b Ratio of AuI species and seed particles in the reaction medium.
c Particles with different shapes, Fig. 7.
d TEM images, Fig. 5.
e There was total precipitation of the particles, leading to a clear solution.
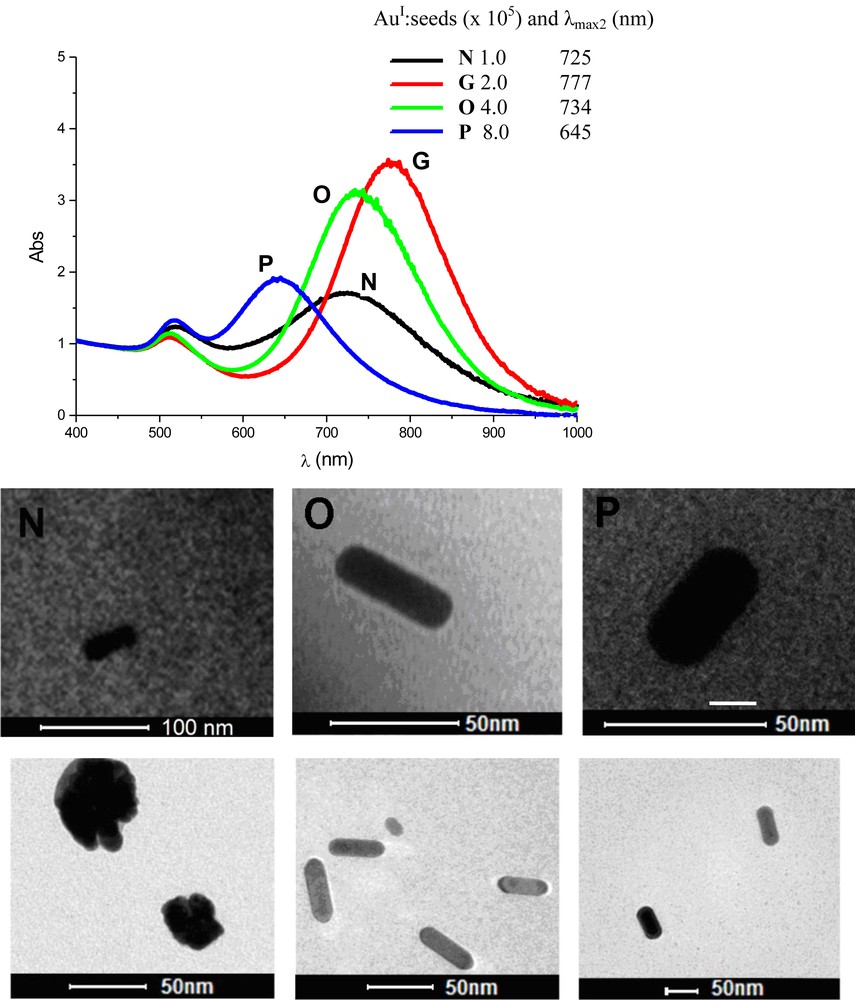
Absorption spectra of the colloids containing gold nanorods prepared with different amounts “monomers” in the reaction medium. All spectra were obtained 24 h after rod formation. Samples N, G, O, and P were prepared from growth solutions with AuI concentration of 0.32, 0.64, 1.28, and 2.56 mmol/L, respectively. TEM images of gold nanorods obtained from samples N, O, and P. Volume of seed solution added: 120 μL.
In this study, we determined that the concentration of the solution employed is in fact important, since the particles are different if the concentrations are different. This demonstrates that diffusion effects play a role in the particle formation process. Overall, we observed that, at higher concentrations, the particles formed were generally larger and with a lower aspect ratio. This is a curious result. We expected that, having the same number of particles formed, since the same amount of seed was added, we should expect that particles formed with a greater should have a reduced width; however, this is not the case. To explain such an incongruence, two events are possible: (i) at higher concentrations, some seed particles do not develop or (ii) in diluted conditions, part of the “monomers” do not have access to the surface of the growing particles. We are carrying out additional experiments to get more information in order to understand such tendencies. However, we suggest that at higher concentrations, some seed particles are blocked by the high concentration of CTAB in the medium, thus, prohibiting the development of some seed particles into AuNRs. This results in more “monomers” available to those seed that do develop, leading to larger particles.
4 Conclusion
Although the synthesis of AuNRs is already considered a consolidated subject, some aspects of their preparation still generate discussion. In this work, we verified that the synthesis of anisotropic AuNPs via the seed-mediated method is quite susceptible to the reaction conditions adopted. Several variables, such as reagent concentrations and the molar ratios between them are important aspects to be considered to tune the synthesis of the desired particles. Different results are obtained with reactions with the same molar ratio between the reagents, but under different concentrations. With the method of synthesis employed here, the desired particles were formed between 2 and 4 h and were stable for around 2 days, where after Ostwald ripening began to govern the growth process. To obtain good quality AuNRs in good yields, without enrichment, we suggest keeping the growth-driving agent/gold molar ratio between 200 and 100 and the AuI/seed particle ratio between 10.0 × 105 and 2.0 × 105. Nevertheless, one must take in account the concentration of the reagents in the final solution within specific ranges. For example, even keeping the AuI/seed particle molar ratio at reasonable values, in relatively highly concentrated reaction solutions, some seed particles do not develop. However, in diluted conditions, some of the “monomers” do not have access to the surface of the growing particles, since the seed particles are blocked by the high concentration of CTAB in the medium.
Acknowledgments
The authors thank CNPq, CAPES, and FAPEAL for partial financial support and for their research fellowships.