1 Introduction
Volatile organic compounds (VOCs) are known as one of the major contributors to atmospheric pollution. Their anthropic releases are particularly important and have some consequences in health, environment, and construction materials.
An important part of these VOCs are single-aromatic molecules, which are principally represented by the BTEX class (benzene, toluene, ethylbenzene and xylenes). Indeed, these compounds are widely used in all industrial sectors: fuel additives, solvents (paints, varnishes, lacquers, inks, adhesives and glues), cleaning or extracting agents, synthesis precursors (production of explosives, pesticides, drugs and polymers). In addition to their highly inflammable and explosive character, BTEX compounds are highly volatile and have a high mobility in air, soil and water. Being very irritating and highly toxic (disorders of the digestive, respiratory, nervous and neurological systems), they can quickly contaminate and poison an ecosystem.
Therefore, it is necessary to set up techniques for reducing BTEX emissions. Nowadays, catalytic oxidation represents an economical and environmental alternative to the thermal oxidation, the latter being the most common in the industry. Indeed, the use of catalysts allows a drastic reduction of the process temperature (200–500 °C), which prevents the formation of toxic by-products (NOx, dioxins) and reduces the energetic cost of the process.
In order to treat gaseous effluents loaded in BTEX from industry, the catalytic total oxidation of toluene was here studied using several Pd-based materials. These materials have been selected by taking into account their cost, activity, stability (thermal, mechanical and chemical), and it can be easy to produce this material at a large scale. Concerning supports, titanium oxide and HY Faujasite show interesting performances in the catalytic oxidation reactions of BTEX [1–4]. Then, cerium oxide is known for the treatment of automobile exhausts, but this material also shows good performances as a support for BTEX oxidation [5,6]. Finally, aluminium oxides were the most common support in catalytic process, especially α-Al2O3 and γ-Al2O3. They present some interesting properties, like a high thermal stability for α-Al2O3 or a high specific surface area with acidic properties for γ-Al2O3, and also present good performances as a support for BTEX oxidation [7–10]. Palladium was chosen as the active phase seeing that it is the most suitable noble metal in an oxidizing environment. In fact, palladium is more resistant than platinum to sintering, vaporizing metal species and poisoning [11–13]. These properties can be partly explained by the reversible transition between the two phases of palladium, Pd0/PdIIO, which has been demonstrated by Cordi et al. [11]. Moreover, palladium and platinum show very close performances for VOCs catalytic oxidation [14,15]. Therefore, palladium is a good compromise between performance and cost. Thus, palladium was impregnated to 0.5 wt% on α-Al2O3, TiO2, HY Faujasite and CeO2. A commercial catalyst (0.5 wt% Pd/γ-Al2O3) was selected as a reference.
As all destructive techniques for treating VOCs emissions (thermal oxidation, plasma, biological treatment…), catalytic oxidation can generate some by-products that are intermediate compounds more difficult to oxidize than the substrate and can be potentially released into the atmosphere. Some studies have demonstrated the formation of its intermediaries, particularly for oxygenates [16–18] and aromatics [19–21] compounds. Some of these compounds are known to interact with cell macromolecules, like DNA. They can therefore exert their toxicity, and induce some pathologies [22]. Recent concern has centred on the effects of continuous exposure to low concentrations of VOCs, like toluene and benzene, both occupationally and environmentally. Some of them have for a long time been recognised as carcinogenic for humans. For example, benzene, the major by-product produced by toluene catalytic degradation, is known to induce acute myeloid leukaemia (AML). In a previous work, we showed that A549 cells were a good cell model for the determination of benzene and toluene effects on human lung cells. Mutation hotspots in the tumour suppressor gene TP53, which are the most common genetic alterations involved in human cancer, were the same in AML and in A549 in vitro exposed to benzene [23]. Consequently, several questions can be asked. Are all by-products removed when the conversion of the initial VOC is total? If not, is it possible to find a compromise between efficiency and economic viability? Finally, can they have toxicological or ecotoxicological impacts? So, an important part of this work concerns the oxidation of toluene, specially the identification of toluene by-products. In a first time, light-off curves were determined by microGC analysis, which are suitable for fast and sensitive analyses. A coupling with a quadrupole mass spectrum allowed for a more complete identification of by-products. In a second time, coupling the catalytic process with a cell exposure system permitted us to study the impact of the by-products on human lung cells by measuring the gene expression of xenobiotic metabolizing enzymes (XMEs) involved in the biotransformation of the organic compounds. Coupling an air–liquid interface (ALI) system, called Vitrocell®, to a catalyst test was performed for the first time during this study.
2 Materials and method
2.1 Preparation of supports
The ceria support was prepared by a precipitation way. A solution of Ce(NO3)3·6H2O was added dropwise to a NaOH solution with a molar ratio of 5 under stirring during 3 h. The suspension was left under agitation during 2 h at ambient temperature. Then, the suspension was filtered and washed 6 times with 200 mL of hot deionized water (∼ 60 °C). The solid was dried 24 h at 100 °C and calcined for 4 h at 500 °C (heating at 1 °C/min) under an air flow (2 L/h).
The titania support was prepared by a sol–gel way. A 15-wt% cetyltrimethylammonium bromide (CTMABr) micellar solution was added dropwise to a solution of sulfuric acid (pH = 2) under magnetic agitation during 3 h at 40 °C. Then, a solution of titanium (IV) tetraethoxide was added dropwise with a molar ratio CTMABr/Ti4+ of 0.33. The agitation was maintained for 1 h at 40 °C before the addition. Afterwards, the solution was placed in a Teflon sheath that was inserted in a stainless steel autoclave. This one was placed during 48 h in an oven at 60 °C for hydrothermal treatment. The surfactant was eliminated by Soxhlet extraction with ethanol. The solid was dried 48 h at 60 °C and calcinated 4 h at 400 °C (heating at 1 °C/min) under an air flow (2 L/h).
HY Faujasite zeolite was prepared by ionic exchange. A commercial NaY Faujasite zeolite (Si/Al: 2.7; Sigma–Aldrich) was slurried in a solution of ammonium nitrate 2.0 mol/L with a molar ration NH4+/Na+ of 20. The suspension was maintained 18 h at 80 °C under magnetic agitation. Then, the suspension was filtered and washed with hot deionized water (∼ 60 °C) to a neutral pH. These operations were repeated three times. Then, the solid was dried one night at 100 °C and calcined for 4 h at 400 °C (heating at 1 °C/min) under an air flow (2 L/h).
For the α-Al2O3, a commercial powder was chosen (Acros Organics).
2.2 Palladium impregnation
Palladium was deposed on the support (α-Al2O3, TiO2, CeO2 and HY Faujasite) by an aqueous impregnation method (0.5 wt% Pd). The solid was suspended in an appropriate volume of a palladium nitrate solution (0.25 g/L). The suspension was maintained during 18 h at 60 °C. Then, water was removed by a rotary evaporator. The solid was dried one night at 100 °C before calcination at 400 °C (heating at 1 °C/min) under an air flow (2 L/h).
A commercial 0.5 wt% Pd/γ-Al2O3 catalyst (Acros Organics) was chosen to complete the study.
2.3 Characterization of the catalysts
The BET surface area was measured by nitrogen adsorption at –196 °C in a Thermo-Electron QSurf series Surface Area Analyzer apparatus. The sample was degassed before measurement at 130 °C under a helium flow. Adsorption was made with a gas mixture of 30% N2–70% He. The desorption of gaseous N2 was quantified with a thermal conductivity detector.
An amount of 50.0 mg of powder were dissolved in 5 mL of aqua regia (HNO3/HCl 1:2) under microwave irradiation during 30 min (CEM, model MARSXpress). Then, the solution was extended to 50.0 mL with ultrapure water and filtered with a 0.45-μm cellulosic micro-filter. The analysis was performed with an ICP-OES (Thermo, model ICAP 6300 Duo).
2.4 Catalytic test
The total oxidation of toluene was studied in a conventional fixed-bed reactor loaded with 100 mg of catalyst. A toluene/air mixture was generated with a saturator in order to obtain 1000 ppm in a flow of 100 mL/min. Tests were made between 50 and 400 °C with a temperature ramp of 1.5 °C/min. Catalysts were pre-treated at 200 °C (heating at 1 °C/min) during 2 h under an air flow (2 L/h). For the impregnated catalysts, they were reduced for 2 h at 200 °C (heating at 1 °C/min) under a dihydrogen (5.0) flow (2 L/h). The organic compounds were analysed by gas chromatography with a CP-4900 microGC (Agilent) coupled with a Pfeiffer-Vacuum Omnistar Quadrupole Mass Spectrometer (QMS-200). The mass spectrometer is configured with a measurement mode MID (Multiple Ion detection) that is adapted for the detection of compounds trace. CO and CO2 were quantified with an infrared analyser (ADEV 4410 IR).
Toluene conversion was calculated considering products and by-products and as a function of the number of carbons for each compound:
The catalytic activity was calculated at a toluene conversion of 20% and considering a plug-flow reactor:
Catalytic performances were compared by considering T50 and T100, which respectively correspond to the temperatures at which 50 and 100% of toluene were converted. The value of T100 was chosen in order to achieve total oxidation for a catalytic industrial application.
2.5 Cells, culture conditions and coupling of the exposure system of the cells
Toxicological studies were made by coupling the catalytic process with an air–liquid interface (ALI) exposure system Vitrocell® in order to expose human lung epithelial adenocarcinoma cells A549. In vitro toxicological studies involve the direct or indirect exposure of human cells to xenobiotics, and should be carried out under conditions resembling the in vivo situation as far as possible. Among the cell exposure systems, the ALI system appears as the best compromise to expose cells to gaseous mixtures, because it allows the control of the level of exposure during all the exposure period. A549 cells were cultured in sterile plastic flasks (Corning®, Fisher Scientific), in a minimum essential medium (MEM) supplemented with 5% (v/v) of fetal bovine serum, 1% (v/v) l-glutamin and 1% (v/v) penicillin and streptomycin (Invitrogen-Life Technologies). These cells are known to activate VOCs using XMEs [22]. Cells were incubated at 37 °C, in a 5% CO2 and 100% humidity atmosphere until 80% of confluency. Then, cells were washed twice with PBS and dissociated with trypsin + EDTA (Gibco Invitrogen) at 37 °C during 3 min. The cells were collected by centrifugation and counted with a Cellometer®. Four hours before ALI exposure experiments, 5 × 105 cells were seeded in Transwell insert with a surface area of 4.7 cm2 in MEM supplemented with 1% (v/v) of l-glutamin.
Three culture modules, each one containing three Transwell inserts with A549 cells maintained at 37 °C, were exposed in parallel to different gases:
- • gas from the catalytic exhaust without dilution;
- • gas from the catalytic exhaust with a dilution of 10% in purified air;
- • purified air (control).
On the one hand, the 10% dilution exposure mimics a quite realistic dilution in the atmosphere. On the other hand, this helped us to evaluate the dose–response relationship of catalytic exhausts. Exposures are performed at fixed catalytic conditions during 1 h. These conditions correspond to the beginning of 100% toluene total conversion (T100). After 1 h of exposure to catalytic exhausts, the Transwell inserts containing cells were rinsed with PBS and supernatants were recovered. Both were frozen at –80 °C until use.
2.6 Toxicological analysis
The cell membrane integrity of A549 cells was evaluated by measurement of extracellular released lactate deshydrogenase (LDH) in the culture medium, according to the manufacturer's instructions (Roche). Briefly, 100 μL of each cell-free culture medium were transferred in triplicate into a 96-well plate. Then, 100 μL of LDH-assay reaction mixture were added to each well. After 30 min of incubation at 37 °C in the dark, the optical density at 490 nm was measured using a microplate reader.
Total RNA extraction from exposed or unexposed cells and cDNA synthesis from mRNA were carried out using TaqMan® Gene expression Cell-to-Ct kit (Ambion, Invitrogen-Life Technologies), following the manufacturer's instructions. Gene expression of xenobiotic metabolizing enzymes (XMEs) (i.e. CYP2E1, CYP2S1, CYP1A1, CYP1B1, EPHX1, NQO1) and 18S rRNA as housekeeping gene were quantified using TaqMan® Gene Expression Assay (Applied Biosystems) combined with TaqMan® specific primers sets (CYP2E1; Hs00559368_m1, CYP2S1; Hs00258076_m1, CYP1A1; Hs01054797_g1, CYP1B1; Hs02382916_s1, EPHX1; Hs01116806_m1, NQO1; Hs02512143_s1 and 18S rRNA; Hs99999901_s1) on a 7500 Fast Real-Time PCR System. These XMEs play a major role in the biotransformation of organic compounds, like VOCs as expected, but also of heavier chemicals, like polycyclic aromatic hydrocarbons (PAHs).
After 40 cycles of amplification, each gene expression was determined using cycle threshold (Ct) measured by Sequence Detection Software v2.0.3 and relative quantification (RQ) between exposed and untreated cells was achieved for each exposure using the following calculation: RQ = 2−ΔΔCt (where ΔΔCt = ΔCtexposed – ΔCtunexposed and ΔCt = Cttarget gene – Ct18S). RQ corresponds to the comparison of exposed cells versus unexposed cells (i.e. purified air). The results are expressed as median values of triplicates.
3 Results
3.1 Catalytic results
Table 1 displays the specific surface areas and the palladium content of the catalysts. These different catalytic materials have been studied for the catalytic total oxidation of toluene. Light-off curves of supports have been determined and are reported in Fig. 1. It could be observed (Fig. 1, Table 2) that these materials have a catalytic activity without palladium. HY Faujasite and TiO2 have similar activity values with T50 (temperatures at which 50% of toluene was converted) of 302 and 310 °C, respectively. With a T50 of 233 °C, CeO2 shows the better performance when the conversion is above 80%. On the contrary, alumina shows the worst performance; this support is only active over 350 °C and conversion does not exceed 75% at 400 °C.
Characteristics of catalysts used.
Pd/α-Al2O3 | Pd/TiO2 | Pd/HY | Pd/CeO2 | Pd/γ-Al2O3 | |
BET surface area (m2/g) | 1 | 252 | 900 | 93 | 252 |
Palladium content (wt%) | 0.48 | 0.41 | 0.46 | 0.40 | 0.40 |
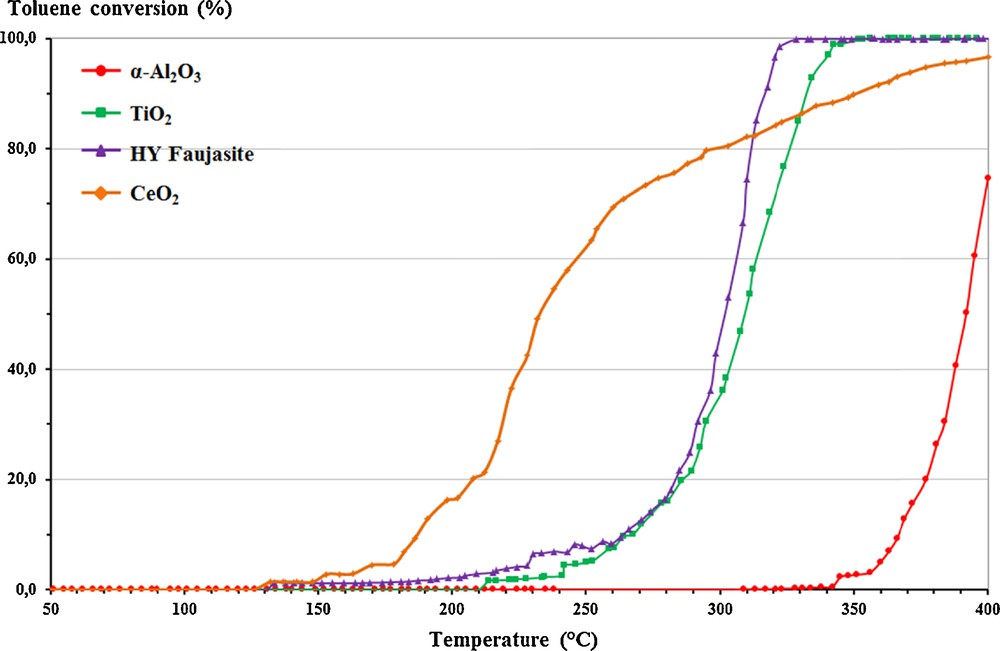
(Colour online.) Light-off curves of the supports.
T50, T100a, and activity of catalysts for the catalytic oxidation of toluene.
Support | Impregnated support | ||||
T50 (°C) | T100 (°C) | T50 (°C) | T100 (°C) | Ai (mol/(m2·h)) | |
α-Al2O3 | 392 | > 400 | 218 | 233 | 2.84 × 10−7 |
TiO2 | 310 | > 400 | 259 | 285 | 1.29 × 10−9 |
HY | 302 | 334 | 222 | 242 | 3.18 × 10−10 |
CeO2 | 233 | > 400 | 197 | 235 | 3.34 × 10−9 |
Pd/γ-Al2O3 | – | – | 179 | 200 | 1.27 × 10−9 |
a Temperatures at which 50 and 100% of toluene, respectively, were converted.
The toluene conversion curves for the impregnated supports have been determined, as well as for the commercial catalyst (Fig. 2). Table 2 displays the T50 and T100 of unimpregnated and impregnated supports. For all samples, Pd impregnation on supports increases the performance of materials, as expected. The commercial catalyst, Pd/γ-Al2O3, shows the best performance in terms of T50 and T100 at 179 °C and 200 °C, respectively. However, as regards the activity value (Table 2) obtained for the catalysts at a toluene conversion of 20%, it could be observed that the catalyst Pd/α-Al2O3 possesses the best activity.
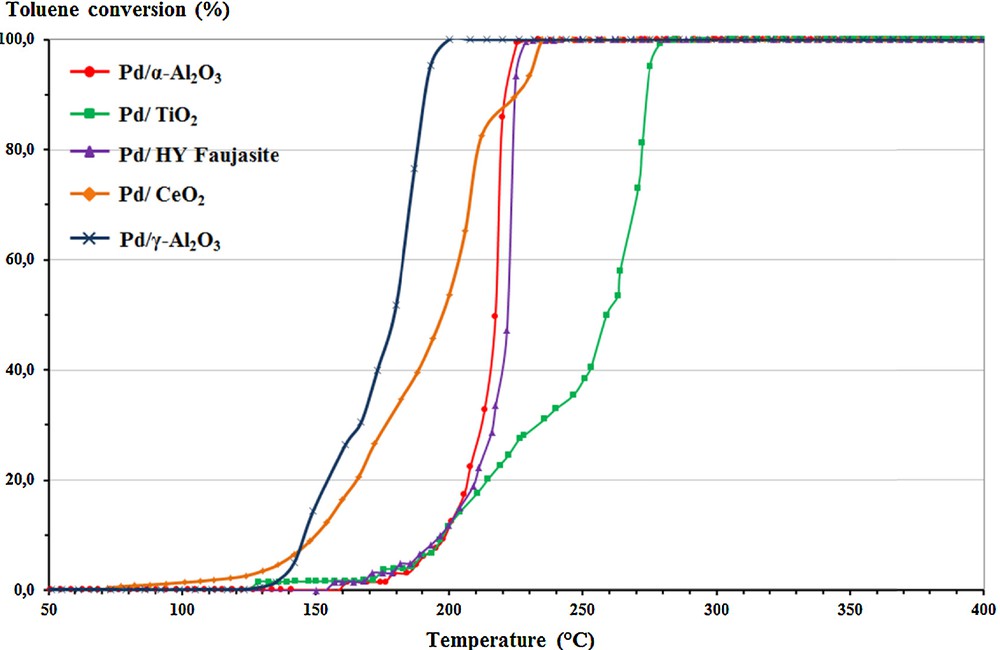
(Colour online.) Light-off curves of the catalysts loaded with 0.5 wt% of palladium.
3.2 Identification of by-products
3.2.1 MicroGC analysis
Lars and Andersson [19] have studied the formation of the by-products generated in the catalytic oxidation of toluene. With the 23 products observed by GLC–MS, they proposed three reaction mechanisms describing the catalytic oxidation of toluene as a function of the initiation mode. However, the study had been made under static conditions and promoting partial oxidation. In dynamic conditions, the knowledge of the generated by-products is limited for VOCs total catalytic oxidation. In order to define the nature of by-products, this study was performed.
Analysis by microGC, which is adapted for quick (less than 4 min) and sensitive analysis, has allowed the detection of the majority of the oxidation intermediates. Benzene was identified as the main by-product resulting from toluene total oxidation. Its toxic properties, particularly its carcinogenic properties, have been known for many years. Consequently, it is important to know under which conditions and in which amounts this compound may be issued by the catalytic process. Benzene could be quantified by microGC, emission profiles have been determined according to toluene conversion and working temperature for each catalyst. Fig. 3 shows a general profile of the benzene-produced amount as a function of temperature. Benzene production begins at the same temperature as toluene conversion. The issued quantity increases rapidly until it reaches a maximum emission, after which the issued amount of benzene decreases slowly. The benzene emission profile may be characterized by four parameters, which are also illustrated in Fig. 3:
- • Ti: the temperature at which a by-product is detected (°C);
- • Tmax: the temperature at which the amount of the by-product is the largest (°C);
- • Qmax: the maximum observed amount of the considered by-product (ppm);
- • Tf: the temperature at which the by-product is totally oxidized (°C).
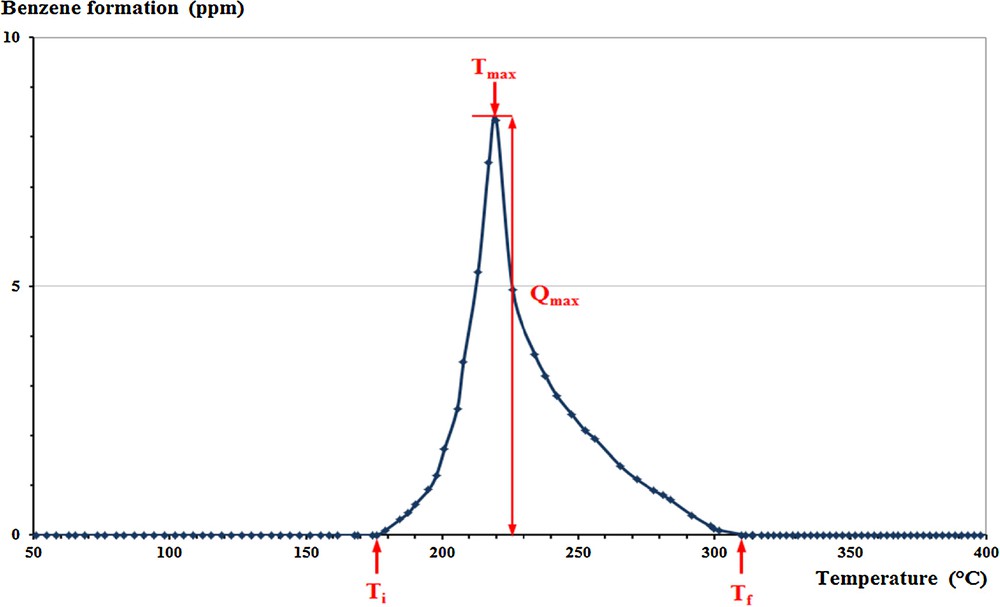
(Colour online.) Typical profile of benzene emission and its characteristic parameters.
In Fig. 4, the dashed lines correspond to benzene production as a function of the toluene light-off curve and temperature for the four impregnated catalysts and the commercial one. The results show quite similar benzene emission profiles for all the catalysts, and this despite the nature of the support. Only Pd/TiO2 shows a different profile, with a particularly intense emission peak. Table 3 reports the values of the four parameters established to describe the benzene profiles: Ti, Tmax, Qmax and Tf. The data show a similar magnitude of Qmax for the catalysts Pd/α-Al2O3, Pd/HY, Pd/CeO2 and Pd/γ-Al2O3 with values ranging between 7 and 14 ppm. However for Pd/TiO2, the emission peak is more significant with a Qmax value of 43 ppm. As regarding the values of Ti, Tmax, and Tf, the results are quite different for each catalyst and no trend can be evidenced.
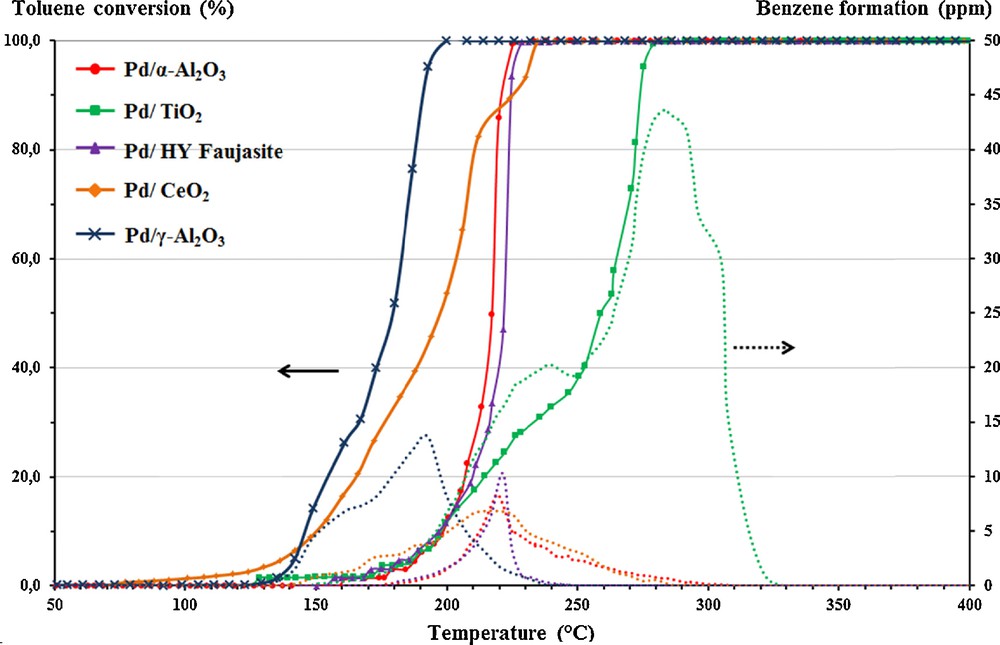
(Colour online.) Toluene conversion and production of benzene versus temperature for the impregnated catalysts.
Parameters characterising the emission profiles of benzene.
Ti (°C) | Tmax (°C) | Qmax (ppm) | Tf (°C) | R (°C) | P (°C) | X (%) | |
Pd/α-Al2O3 | 176 | 220 | 8 | 300 | 124 | 67 | 86 |
Pd/TiO2 | 150 | 285 | 43 | 325 | 175 | 40 | 100 |
Pd/HY | 182 | 222 | 10 | 249 | 67 | 7 | 47 |
Pd/CeO2 | 142 | 212 | 7 | 298 | 156 | 63 | 83 |
Pd/γ-Al2O3 | 129 | 193 | 14 | 244 | 115 | 44 | 95 |
For a better understanding of the by-product generated profile, the values of the range emission of benzene (noted R) and its persistence (noted P) were evaluated then. The first term, R, can be calculated as the difference Tf – Ti. The second term, P, can be considered the temperature that must be added to the T100 of toluene to have a total degradation of toluene and benzene. The persistence can be calculated by the difference Tf – T100. The value of toluene conversion X corresponding to the maximum emission of benzene was also determined. The values of R, P, and X are reported in Table 3. Regarding the emission range, this value is relatively large, with an average of 126 °C. Pd/HY is especially noteworthy, with a R value of 67 °C. Concerning the persistence, P values clearly show that benzene is not removed when toluene conversion is complete. So, it is necessary to increase the working temperature for all catalysts in order to completely remove toluene and benzene. This point is particularly important because an increase in the working temperature has a direct and non-negligible impact on the operating cost of the treatment unit on an industrial scale. The catalyst Pd/HY stands out again with a P value of only 7 °C. In view of these two points, the catalyst Pd/HY, which is not the most active, is the most selective catalyst concerning benzene emissions. Finally, if we consider the X values, the data show that benzene is mainly issued for high conversions in toluene.
A second major by-product evidenced in this study is carbon monoxide. It was also detected during the catalytic oxidation of toluene. It has been quantified and emission profiles of carbon monoxide have been traced. These profiles can be described in the same manner as those of benzene. Fig. 5 shows the toluene light-off curve and carbon monoxide production as a function of temperature for the five catalysts. Previously, it could be observed that the emission profiles of benzene are fairly homogeneous. Concerning the profiles obtained for CO, heterogeneity is revealed. Indeed, the values of Qmax, given in Table 4, are relatively high for the Pd/TiO2 and Pd/HY catalysts with 71 and 117 ppm, respectively. On the contrary, the catalyst Pd/α-Al2O3 has a very low emission peak with a Qmax of 5 ppm. As for catalysts Pd/CeO2 and Pd/γ-Al2O3, they show no CO emission. Regarding the range emission, the R values are generally lower than for benzene. Regarding persistence, P values indicate that carbon monoxide is completely oxidized before the total conversion of toluene is reached. So, it can be concluded that carbon monoxide has no impact on the working temperature for a total conversion of toluene. These two points may be explained by the fact that palladium is a suitable metal for the oxidation of carbon monoxide [11,13].
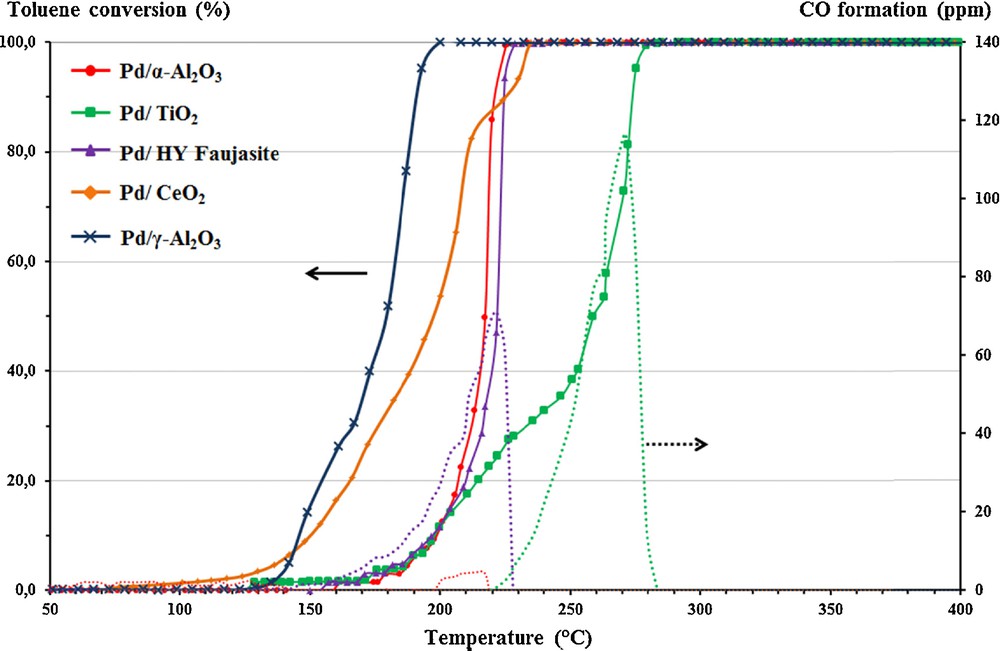
(Colour online.) Toluene conversion and production of carbon monoxide versus temperature for the impregnated catalysts.
Parameters characterising emission profiles of carbon monoxide.
Ti (°C) | Tmax (°C) | Qmax (ppm) | Tf (°C) | R (°C) | P (°C) | X (%) | |
Pd/α-Al2O3 | 198 | 213 | 5 | 220 | 22 | –13 | 33 |
Pd/TiO2 | 222 | 271 | 117 | 284 | 62 | –1 | 73 |
Pd/HY | 125 | 222 | 71 | 229 | 104 | –13 | 47 |
Pd/CeO2 | – | – | 0 | – | – | – | – |
Pd/γ-Al2O3 | – | – | 0 | – | – | – | – |
3.2.2 Mass spectrometry analysis
MicroGC analysis has helped highlight the role of benzene, which is considered the main by-product of the catalytic oxidation of toluene. But this device does not allow the detection of molecules below the ppm level. Thus, molecules emitted into much lower concentrations can be detected. For this reason, mass spectrometer was coupled with microGC, allowing analysis of by-product traces. The apparatus, configured for dynamic analysis, can follow the evolution of the characteristic fragments of molecules that interest us as a function of temperature. These fragments were chosen primarily based by-products observed in the work of Lars and Andersson [19]. Characteristic fragments of C1–C6 paraffins and some common families of polycyclic aromatic hydrocarbons (C10H8, C14H10, C18H12, C22H14) were also selected.
Table 5 lists the molecules that were identified by mass spectrometry. In general, the same compounds are observed for all the catalysts. On the one hand, benzyl alcohol, benzaldehyde, benzene, 1,4-benzoquinone and maleic anhydride were identified. These compounds are derived from the main way toluene oxidation proposed by Lars and Andersson [19] and given in Fig. 6. Phenol and hydroquinone were not detected by mass spectrometry; this may be explained by a high reactivity of these molecules. As for benzoic acid, it cannot be identified with certainty, as its characteristic fragments are common with those of the previous molecules. On the other hand, 2-methylmaleic anhydride, 2-benzofuran-1,3-dione (phtalic anhydride), methyldiphenylmethane and dihydroanthracene were identified. These molecules are characteristics of the second way of toluene oxidation, which comes from an oxidative coupling of two molecules of toluene [19]. This results in the formation of methyldiphenylmethane and diphenylethane. Moreover, many compounds from this way are isomers of formula or position. Thus, all these molecules display the same fragmentation, which is also common with the monoaromatics of the main way. This is why so few by-products of this pathway can be identified. These observations, and the fact that benzene is the only by-product observed by microGC, confirm that the predominant way for the catalytic oxidation of toluene is the first way described by Lars and Andersson [19], shown in Fig. 6.
Traces of by-products observed by mass spectrometry.
By-products observed | Pd/α-Al2O3 | Pd/TiO2 | Pd/HY | Pd/CeO2 | Pd/γ-Al2O3 |
Hydrogen | √ | √ | √ | ||
Methane | √ | √ | √ | √ | √ |
Furan | √ | √ | √ | √ | √ |
Maleic anhydride | √ | √ | √ | √ | √ |
2-Methylmaleic anhydride | √ | √ | √ | √ | √ |
Benzene | √ | √ | √ | √ | √ |
Benzaldehyde | √ | √ | √ | √ | √ |
Benzyl alcohol | √ | √ | √ | √ | √ |
Benzoquinone | √ | √ | √ | √ | √ |
2-Benzofurane-1,3-dione | √ | √ | √ | √ | √ |
Dihydroanthracene | √ | √ | √ | √ | √ |
Methyldiphenylmethane | √ | √ | √ | ||
Naphthacene | √ | √ | √ | √ | √ |
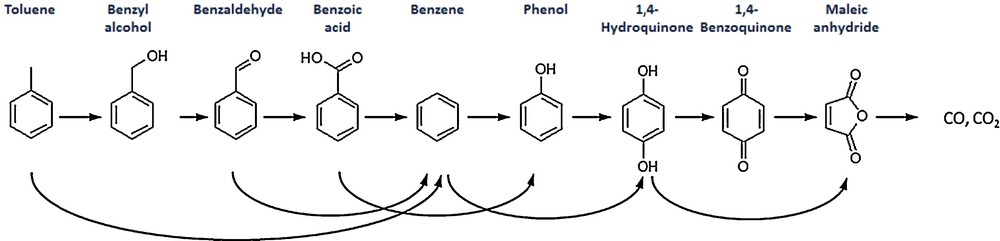
Main way of toluene oxidation proposed by Lars and Andersson [19].
Mass spectrometry has also allowed the identification of other molecules. On the one hand, methane and the hydrogen could be identified. The first may be explained by the cracking of the methyl function of toluene, leading to benzene. The formation of hydrogen can be explained by the oxidation of benzyl alcohol in benzaldehyde. On the other hand, a response was observed concerning the fragments m/z 43, 57, 71 and 85. These are characteristic of C3–C6 paraffins. Their presence can be explained by the opening and catalytic cracking of the aromatic ring. Finally, a response was observed concerning the fragments m/z 113 and 114, which are characteristic of C18H12 PAHs, such as naphthacene, chrysene, benzanthracene or benzo(c)phenanthrene.
Nevertheless, it appears that these compounds, which are more reactive than benzene and emitted in smaller amounts, are totally eliminated before benzene. Thus, only benzene appears to be a limiting factor to the catalysts’ performance.
3.3 Toxicological results
With the aim of evaluating the toxicological impact of the by-products, a coupling of the catalytic process with a cell exposure system is performed. Regarding the catalytic results in terms of activity and formation of by-products, the investigation of these toxicological effects using Pd/α-Al2O3 catalyst has been carried out.
In Fig. 7 are reported the profiles of catalyst temperature, toluene conversion and benzene production versus time. After achieving toluene total conversion (T100) at 230 °C, the catalyst's temperature decreases slightly to stabilize at 227 °C. Consequently, the amount of benzene emitted becomes relatively constant, with a value between 4 and 5 ppm during cell exposure.
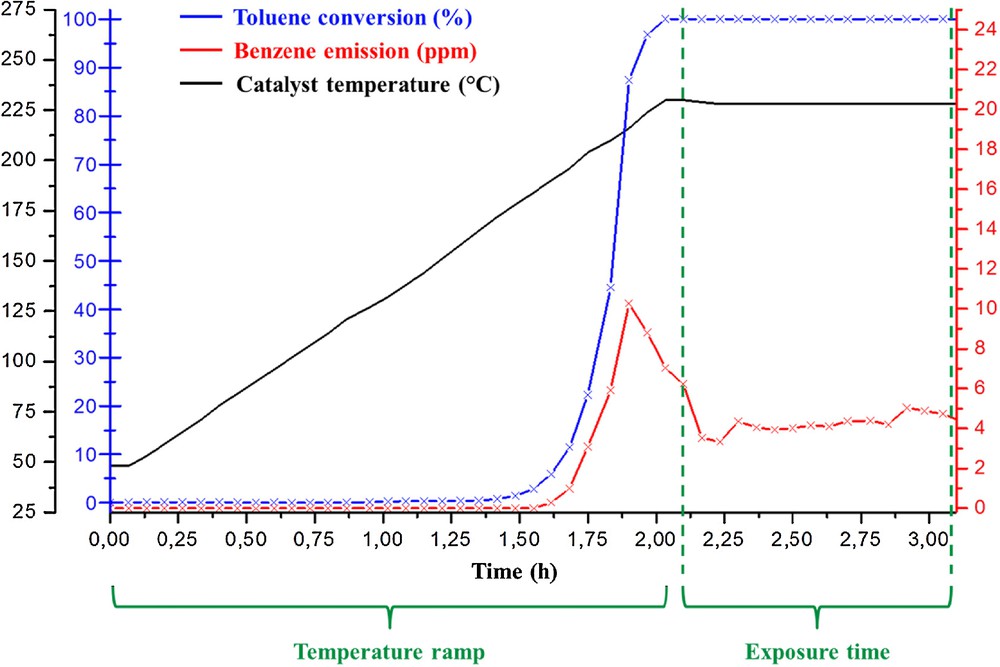
(Colour online.) Temperature of the catalyst and benzene emissions as a function of time during the exposition with the Vitrocell® system for the Pd/α-Al2O3 catalyst.
The study of cytotoxicity did not show any significant increase of the extracellular released LDH level after exposure, despite the presence of carbon monoxide, known for its toxicity. This absence of important cellular death allows us to consider the early underlying physiopathological mechanisms of action occurring before phenotypical cell damages. The mRNA level of the six genes coding for XMEs was checked by qPCR. Their expression was studied in cells exposed to:
- • gas from the catalytic exhaust without dilution;
- • gas from the catalytic exhaust with a 10% dilution in purified air;
- • purified air (control).
When compared to control cells, five genes were significantly upregulated (CYP2S1, CYP1B1, CYP1A1, NQO1 and EPHX1) for the highest dose of exposure (exhaust gas without dilution), while three were significantly upregulated (CYP1B1, NQO1 and EPHX1) for the catalytic exhaust with a 10% dilution in purified air (Fig. 8).
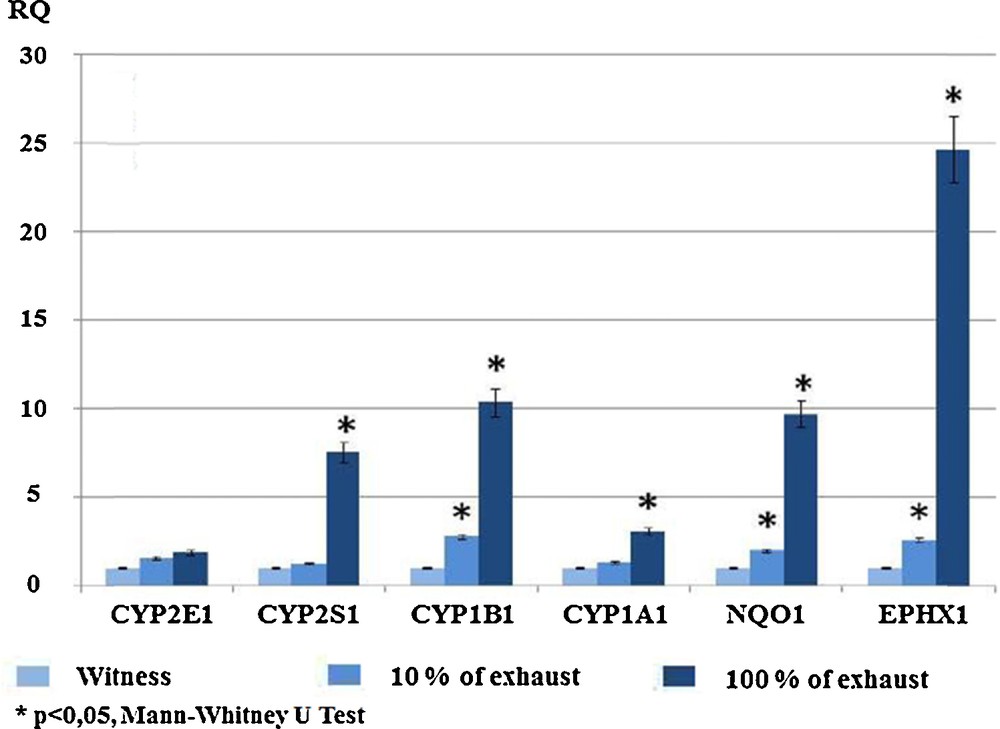
(Colour online.) Gene Expression of xenobiotic metabolizing enzymes in A549 cells exposed for 1 h to purified air (control), or to 10% or 100% of the exhaust gases from the catalytic degradation of toluene by Pd/α-Al2O3 (RQ: relative quantification of the XME mRNA in exposed cells vs unexposed cells).
Firstly, NQO1 and EPHX1 can participate in benzene metabolization. On the one hand, this could be linked to the presence of benzene when the toluene total conversion is achieved and under static conditions. On the other hand, even with a dilution effect when the effluents are out of the process (10% in air), the results indicate a toxic response to benzene.
Secondly, CYP2S1, CYP1B1 and CYP1A1 are mediated by the aryl hydrocarbon receptor (AhR) [24]. This receptor is a ligand-activated transcription factor involved in the regulation of biological responses to planar aromatic hydrocarbons [25]. This receptor, implied in the regulation of XMEs, such as cytochrome P450, has been shown to be induced by PAHs [26,27]. Consequently, the increase in XMEs gene expression confirms the presence of PAHs in the catalytic exhausts in these conditions. However, this does not exclude the presence of other heavier PAHs than C18H12, which has not been detected by chemical methods.
In addition to the necessary compromise to find between performance and cost, toxicological studies show that it is also fundamental to consider the health impacts of catalytic processing units. This increases the relevance of performing a toxicological validation of these systems.
4 Conclusion
This work deals with the identification of the by-products of the catalytic oxidation of toluene. Firstly, microGC analysis coupled with a mass spectrometer allowed detection and identification of many organic compounds resulting from toluene oxidation. However, the main by-product of toluene oxidation is benzene, which can be explained by a low chemical reactivity of this aromatic compound. A typical profile of benzene emission has been described for each one of the catalysts studied, despite the persistence and the temperature range. Moreover, benzene seems to be a factor limiting the performance of catalysts, because it is not completely eliminated when the toluene is totally oxidized (T100). Thus, it is necessary to operate the process at a working temperature higher than T100 in order to obtain a total oxidation of toluene and benzene. Therefore, for an industrial processing unit, it is necessary to select a catalyst that is efficient for the oxidation of VOCs, but also for the oxidation of their by-products, especially when these by-products exhibit toxic properties.
Secondly, the oxidation of toluene was investigated under static conditions in order to expose human lung cells. On the one hand, the results show that the amount of benzene emitted remains relatively stable, between 4 and 5 ppm. On the other hand, the cells show a toxic response partly due to benzene, even when a dilution is performed. This one simulates a quite realistic exhaust dilution in the atmosphere at the output of the treatment process.
This study confirmed that some by-products might be emitted by the catalytic process under certain operating conditions. It is necessary to take into account these by-products emissions in the evaluation of the catalytic performances. Thus, in the case of applications to real industrial effluents, it is necessary to make compromises between performances, operating costs, and potential health impacts. Moreover, this increases the relevance of performing a toxicological validation of these systems.
Acknowledgements
The authors want to acknowledge the French Environment and Energy Management Agency (ADEME) for the financial support of the CORTEA PROBTEX project (1281 C0095), as well as the Nord-Pas-de-Calais Region for the funding of the work and the “Agence universitaire de la Francophonie” for Al Zallouha's master fellowship. The authors also acknowledge the “Centre commun de Mesures” of the “Université du Littoral Côte d’Opale”.