1 Introduction
Erosion is often thought of as a sculptor of landscapes, carving hills and valleys out of the rock of the earth's crust, using water, ice, and wind as the tools. The sculptor metaphor fails several reality tests, however, when one considers that the tools of landscape sculpture (Gilbert, 1877) are weak fluids more likely to caress rock than carve it. Moreover, the sculptor cannot shape different parts of his sculpture at once. The carving of the arm does not simultaneously dictate the shape of the foot. Landscapes, however, do evolve all at once, with the activities in one area affecting, even controlling what happens elsewhere. Because of these spatial linkages or teleconnections, the weathering of any one place in a landscape is imperfectly connected to climate or erosional drivers.
Earth's surface is more than skin deep. The critical zone is the surface boundary layer between the lithosphere and the atmosphere, where atmospheric fluids penetrate into and interact with crustal rocks (Brantley et al., 2007), and this entire zone of interaction can be thought of as the Earth's surface. The surface is thought of as “critical” (National Research Council (NRC), 2001) because it supports life, supplies resources such as water, food, and building materials to humans, and because it has the capacity to unleash hazards such as landslides and floods. While often portrayed as a one-dimensional profile (e.g., Anderson et al., 2007), the critical zone comprises the full three-dimensional surface topography along with the spatially variable subsurface architecture of soils, weathered rock, and porosity distribution, and is pervaded with water and living organisms. When viewed from the three-dimensional landscape perspective, the connection between chemical evolution and erosion is not simple.
Erosion is the loss of mass from the Earth's surface. When one considers mass losses in solution and mass losses due to net removal of solid material (rock or soil), it is clear that the process of erosion is also more than skin deep. Mass losses occur wherever water penetrates and carries dissolved material away, and hence occur throughout the full depth of the critical zone. There are at least two aspects of the connection between chemical weathering and erosion that are important from a landscape evolution perspective.
The first connection between chemical weathering and physical erosion arises from the impact of weathering processes on production of sediment. Weathering progressively weakens rock through fracturing and chemical alteration, thereby, preparing it for eventual transport by some surface process – perhaps stress applied by growth of a tree root, by the furtive clawing of a digging rodent, or by the weight of a mass of soil after a rain storm tips the balance of forces in favor of a landslide. In any case, physical displacement occurs when the available applied stress exceeds the strength of the material. When we enumerate erosion mechanisms, we normally describe a process that exerts the driving stress, such as shear stress due to flowing water, the gravitational stress on a slope, or stress exerted by growth of ice lenses. The actual effectiveness of these mechanisms, however, depends on the strength of the material to resist these drivers, and this is affected by weathering within the critical zone. If weathering processes – both physical and chemical – weaken the rock, it will more easily be entrained into the sediment transport system on the hillslopes, and therefore, more likely transported into streams. Thus, enhanced weathering damage of rock can lead to increased erosion.
The second point to make about the connection between chemical weathering and erosion is that, because evolution of landscapes takes time, landscapes and erosion are rarely in equilibrium with climate (Fernandes and Dietrich, 1997). Lags between climate and base-level drivers of hillslope evolution imply that sediment flux will not be perfectly in phase with the driving force. While these lags have been explored with respect to evolution of topography and sediment fluxes, their impact on chemical fluxes and geochemical evolution of profiles is not much explored. To the extent that chemical weathering processes impact sediment production and hence physical erosion rate, the time it takes geochemical processes to respond to climate and base-level may be significant with respect to the full evolution of the critical zone.
Here, we present a conceptual model of critical zone evolution, using the landscape of Boulder Creek Critical Zone Observatory (BcCZO) in the Colorado Front Range as our inspiration. The distribution of rock types and large topographic gradients in the watershed give rise to different erosion regimes across the watersheds, which vary with climate (Fig. 1). The changes in sediment and water fluxes in turn impose changes in base-level that affect the evolution of surrounding hillslopes over time. Thus, we will see that the erosion response to climate change is not the same everywhere in the watershed, and in fact, erosion and deposition can occur in different regions at the same time in response to the same climate. In addition, lags bring about evolution incited by climate change that play out long after the climatic event.
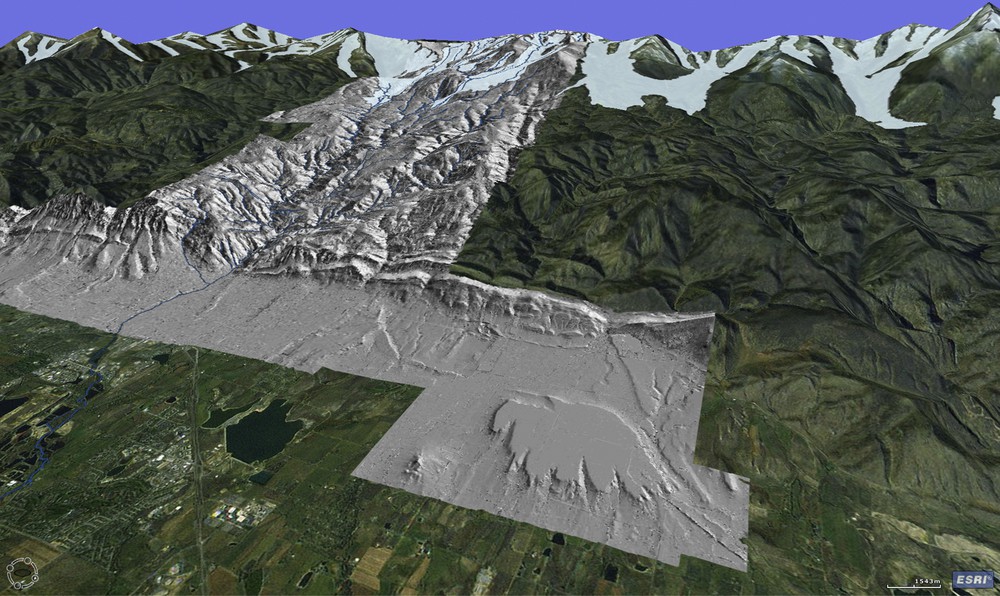
Oblique view of Boulder Creek watershed in Colorado, USA. Gray hillshade from LiDAR topography data (http://opentopography.org/id/OTLAS.032012.26913.1) is draped over a Google Earth image. View is to the west, looking from the High Plains in the foreground, to the high peaks in the distance that define the Continental Divide and the crest of the Colorado Front Range. The abrupt mountain front coincides with the boundary between sedimentary rocks of the Plains and crystalline igneous and metamorphic rocks of the Front Range. Pleistocene glaciers that filled glacially-scoured valleys in the headwaters are shown at their Last Glacial Maximum extent (Madole et al., 1998). Deep canyons stretching upstream from the smooth terrain of the High Plains cut the crystalline rocks. A prominent abandoned fluvial terrace, one of many found on the High Plains, is seen in the LiDAR hillshade in the foreground.
Vue oblique du bassin versant de la Boulder Creek, au Colorado, États-Unis. L’ombre grisée de la colline (données topographiques LIDAR, http://opentopography.org/id/OTLAS.032012.269.13.1) recouvre une image Google Earth. La vue est prise vers l’ouest, depuis les High Plains au premier plan jusqu’aux sommets élevés, à la distance que définissent la Continental Divide et la crête du front de chaîne du Colorado. Le front abrupt de la montagne coïncide avec la limite entre les roches sédimentaires des Plaines et les roches cristallines ignées et métamorphiques de la Chaîne frontale. Des glaciers pléistocènes qui ont rempli des vallées à creusement glaciaire dans la zone des eaux d’amont sont représentés dans leur extension du Dernier Maximum Glaciaire (Madole et al., 1998). Des canyons profonds s’allongeant à l’amont à partir du terrain meuble des High Plains coupent les roches cristallines. Une terrasse fluviale abandonnée saillante, l’une des nombreuses observées dans les High Plains, est visible dans la zone ombrée LIDAR au premier plan.
2 Conceptual model
The BcCZO is built around the idea of understanding how erosion and weathering control the evolution of critical zone architecture (Anderson et al., 2008). Boulder Creek watershed (Fig. 1) provides a natural laboratory for examining erosion and weathering histories and resulting critical zone architectures, because it encompasses several different erosion regimes (Fig. 2). Boulder Creek runs east from the crest of the Colorado Front Range onto the High Plains of western United States (Fig. 1), spanning elevations from ∼4000 to ∼1500 m. The Front Range is one of a number of mountain ranges formed in the compressional tectonics of the Tertiary Laramide Orogeny (Bird, 1998). The orogeny thrust Paleoproterozoic crystalline rocks up against the marine sediments from the Cretaceous seaway. The first order topographic feature of the watershed arises from this lithologic contrast set by tectonic history. That is, the crystalline rocks of the Front Range tower above the soft sedimentary rocks of the Plains, and the two provinces are separated by an abrupt topographic front. As the name suggests, the Plains comprise smooth topography with broad alluvial terraces standing in low relief above the modern rivers. The Front Range contains more rugged terrain cut into crystalline rocks, notably deep canyons gashed upvalley from the Plains, and steep-sided glacial valleys hugging the range crest (Anderson et al., 2006).
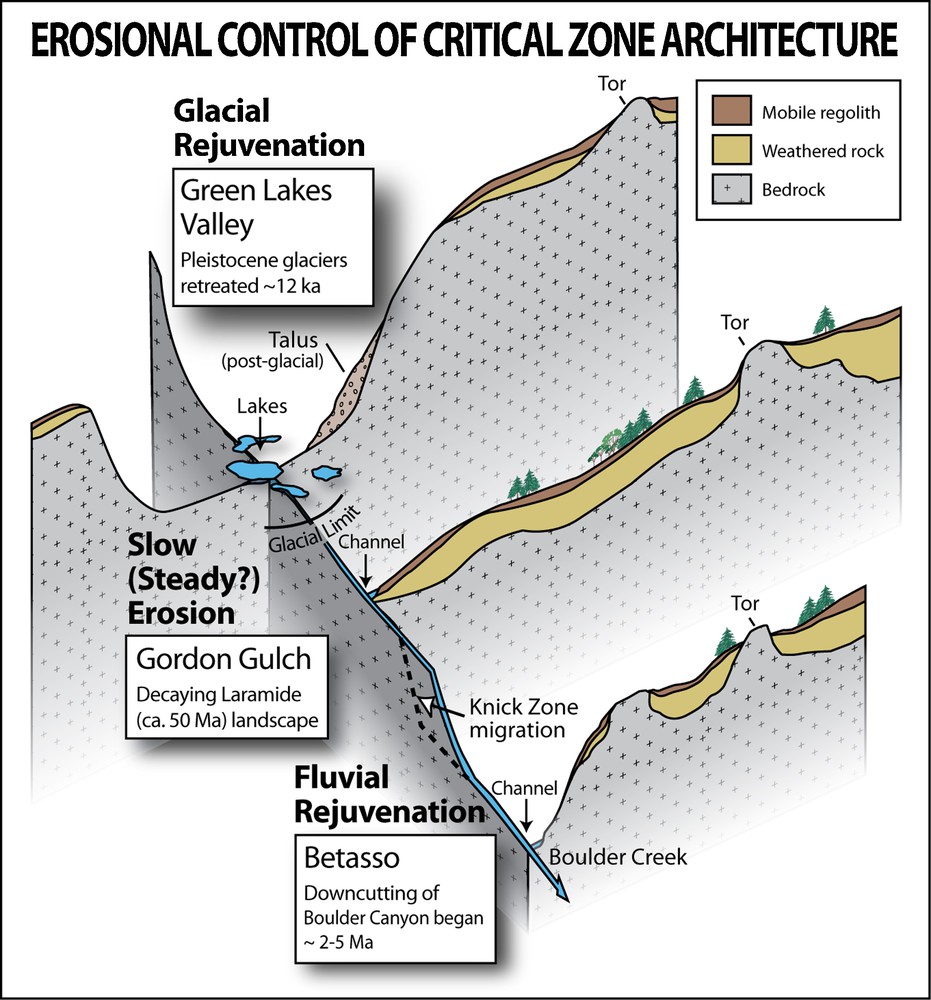
Conceptual figure of influence of differing erosion histories on critical zone architecture in Boulder Creek CZO. This fence diagram illustrates typical valley cross-sections at three points along a typical Front Range stream profile, with examples drawn from specific sites in Boulder Creek. The critical zone is stripped of weathered rock in the headwaters, where glaciers carved U-shaped valley cross-sections in the Pleistocene, typified by Green Lakes Valley at the head of North Boulder Creek. A region of possible steady-state weathering and erosion is found downstream of the glacier limit, as typified by Gordon Gulch, a small tributary to North Boulder Creek. Downstream of prominent knick zones in rivers draining the Front Range, hillslopes have been steepened, and weathered profiles are thin or absent. Betasso, a small tributary to Boulder Creek, displays one stage in the evolution of the critical zone that follows the rapid base-level lowering due to passage of the knick zone. The landscape is also shaped by differing climatology with elevation. Mean annual precipitation and fraction falling as snow increase upstream, and mean annual temperature decreases upstream.
Figure conceptuelle de l’influence d’histoires d’érosion variées sur l’architecture de la zone critique de la Boulder Creek (CZO). Le diagramme illustre des sections transversales de vallées typiques, en trois points le long d’un profil de fleuve typique de la Chaîne frontale, sur des exemples tirés de sites spécifiques de la Boulder Creek. La zone critique est arrachée de la roche altérée dans la zone des eaux d’amont, où des glaciers sculptent, dans le Pléistocène, des sections de vallée en U, caractéristiques de la Green Lakes Valley à la tête de la Boulder Creek nord. Une région où altération et érosion sont dans un état vraisemblablement stable se trouve à l’aval, à la limite du glacier, ainsi que le montre le Gordon Gulch, un petit affluent de la Boulder Creek nord. À l’aval de zones de knick saillantes dans le cas de rivières drainant la Chaîne frontale, les pentes de collines sont plus raides et les profils d’altération sont minces ou absents. Le Betasso, un petit affluent de la Boulder Creek, révèle une étape dans l’évolution de la zone critique, qui suit le rapide abaissement du niveau de base au passage de la zone du knick. Le paysage est également modelé par un climat différant selon l’altitude. La pluviosité moyenne annuelle et la fraction des précipitations tombant sous forme de neige augmentent à l’amont, tandis que la température moyenne annuelle y décroît.
The headwaters of Boulder Creek were glaciated during the Pleistocene, which is manifested in U-shaped valleys and large areas of bare, abraded bedrock surfaces in the valley floors and walls (Fig. 2). Below the glacier limit is the Rocky Mountain surface, a region of low relief with soil mantle and tors (outcrops) coexisting on hillslopes (Fig. 2). This area is a product of steady, unperturbed weathering and erosion since the end of the last phase of uplift in the Laramide orogeny. The Rocky Mountain surface is dissected by river canyons, each with a prominent knickpoint or knick zone at its head. Hillslopes lining the canyons are stripped of weathering profiles, although the mechanism is base-level lowering rather than glacial abrasion (Fig. 2). Hillslopes are steepest and show the least weathering in the knick zone, and become progressively less steep and more weathered with distance downstream from the knick zone, a progression that reflects the evolution of the critical zone following the perturbation of rapid river incision. Finally, on the Plains, topography reflects a bimodal erosion history, in which the rivers either broaden their footprint by lateral planation, or cut into bedrock and abandon the alluvium-mantled terraces (not shown in Fig. 2). This punctuated incision (Dühnforth et al., 2012) is ultimately tied to the headward migration of the knickpoint in the crystalline rocks of the canyons.
In each of these erosional settings, the architecture of the critical zone represents the integrated effects of weathering front propagation and erosional exhumation over time. In the alpine regions of the Front Range, rapid erosion and low chemical weathering front propagation rates under glaciers explain the truncated weathered profiles. Soils in the alpine areas are found only on deposits, rather than from in situ weathering of the bedrock. For instance, moraines, glacial till, talus slopes, and very old diamicton on the ridge tops above recent glacial limits are deposits in which weathering processes produce soils on pre-existing sediments, augmented by eolian inputs (Muhs and Benedict, 2006). The deepest weathering profiles are found on the Rocky Mountain Surface, where they average 7 m in depth (Dethier and Lazarus, 2006). In contrast, on hillslopes lining the fluvial canyons, the extent of weathering and presence of soil is spatially variable. The weathered profile is thinnest and least developed along the river channel and near the knick zone, places where the erosion rates exceed weathering front propagation rates. One can assess development of the critical zone in any of these locations as a one-dimensional problem of weathering processes generating the critical zone, and erosion removing material from the critical zone. Because rates of river incision respond to sediment flux, the production and transport of sediment upstream can influence process rates downstream.
3 One-dimensional view
The critical zone is a region of strong vertical gradients (Fig. 3). Hence, we begin with a vertical profile through the critical zone as controlled by weathering and erosion rates (e.g., Dixon et al., 2009). Its thickness, D, varies through time at a rate set by the rate of advance of a weathering front downward, , and the rate of removal of mass by physical or chemical loss, , as expressed by . Regolith is generally defined as material differentiated from fresh bedrock by any degree of weathering (Taylor and Eggleton, 2001, p. 3), including weathered rock, saprolite, and soil or mobile regolith. Saprolite is isovolumetrically-weathered rock that retains original rock texture, and has not been physically disrupted or displaced, while mobile regolith is disaggregated and displaced material. Rock material moves laterally only in the uppermost layer of regolith, which is therefore called mobile regolith. Mass balance for mobile regolith yields the following expression for variations of the thickness, R, of the mobile layer:
(1) |
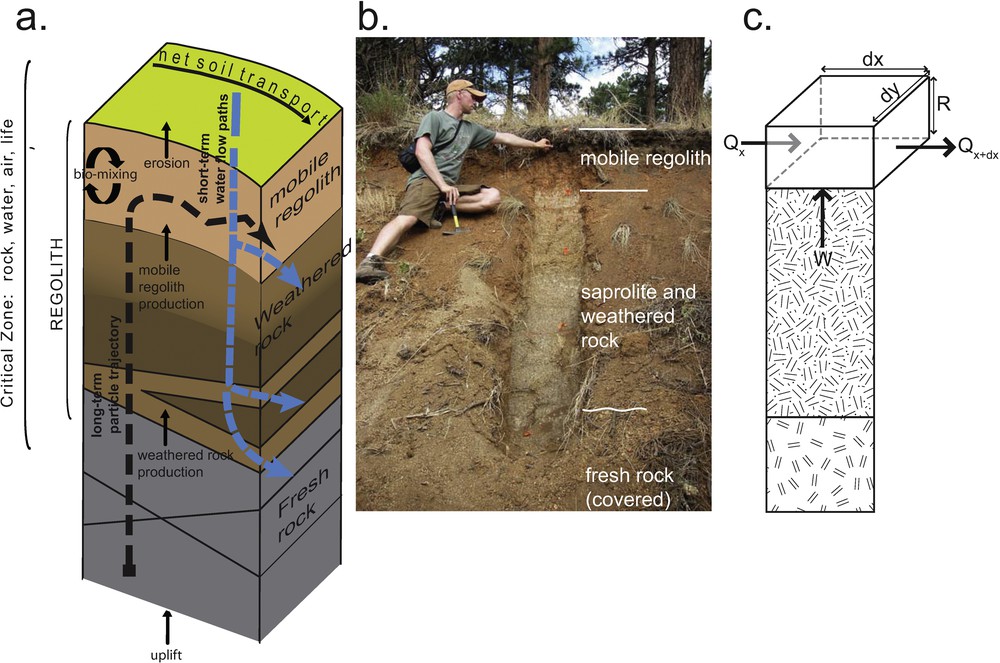
A one-dimensional view of the critical zone. a: block shows how erosion and propagation of weathering fronts bring material through the critical zone, from fresh bedrock to weathered rock (including saprolite), and into mobile regolith. Water dominantly moves downward along vertical paths; b: photo of a weathered profile developed on granodiorite in the Boulder Creek Critical Zone Observatory. A mobile regolith or soil layer can be seen above saprolite. The interface between saprolite and fresh, unweathered rock is covered and not visible here; c: schematic diagram of mass balance for mobile regolith layer in the volume R dx dy, affected by a weathering flux from underlying layers and the flux of mobile regolith through the box sides (block diagram after Anderson et al., 2007; photo by S.P. Anderson).
Vue en une dimension de la zone critique. a : le bloc montre comment l’érosion et les fronts de propagation de l’altération apportent du matériel au travers de la zone critique, depuis la roche mère fraîche jusqu’à la roche altérée (comportant la saprolite) et dans la régolithe mobile. L’eau se déplace surtout vers le bas, le long d’itinéraires verticaux ; b : photo d’un profil développé sur granodiorite dans la zone critique de l’Observatoire de la Boulder Creek. On peut observer une régolithe mobile et un feuillet de sol au-dessus de la saprolite. L’interface entre la saprolite et la roche fraîche non altérée est recouvert et non visible ici ; c : diagramme schématique du bilan de masse pour le niveau mobile de régolithe dans le volume R dx dy, affecté par un flux d’altération à partir des niveaux sous-jacents et le flux de la régolithe mobile au travers des côtés du caisson (bloc diagramme d’après Anderson et al., 2007; photo S.P. Anderson).
The production of debris that moves down hillslopes and feeds the sediment flux in rivers is described by the weathering and transport processes described in Eq. (1). The weathering processes described with are more likely physical disruptions due to agents like roots (Phillips and Marion, 2006), animals (“geomorphic engineers”), or frost (Anderson et al., 2012), than chemical change due to chemical weathering processes, although chemical alteration in the deeper parts of the critical zone undoubtedly influence these processes. Incorporation of material into the mobile regolith layer occurs when a mobilization process (creep, frost heave, tree throw) exerts a stress that exceeds the strength of the rock. Therefore, weathering both chemical and physical that weakens rock below the mobile regolith layer prepares rock for entrainment into the mobile regolith (Anderson et al., 2012; Clarke and Burbank, 2011; Fletcher et al., 2006).
Landscapes maintain a mobile regolith mantle only when Eq. (1) integrated over time yields a non-zero mobile regolith thickness, R. Conditions that enhance the flux of mobile regolith, Qx, may strip away the mobile regolith layer, unless matched by an increase in the mobile regolith production rate, . In a steadily downcutting transport-limited landscape, Eq. (1) applied to slopes yields smooth, convex-up topographic profiles (e.g., Culling, 1965; Gilbert, 1909) and a uniform thickness of regolith. On the other hand, weathering-limited hillslopes will have no simple predictable form.
In the Boulder Creek watershed, the landscape of the Rocky Mountain surface is one where mobile regolith mantles most of the hillslopes, and therefore a balance between flux of mobile regolith, Qx, and mobile regolith production rate, , may exist (Fig. 2). The Qx and terms will be affected by boundary conditions that may be set by processes occurring at a distance. This is what sets up teleconnections – the influence of processes in one place being felt in another place. As we discuss below, these landscape scale linkages set up both spatial differences and temporal lags in landscape response.
4 Landscape scale connections
We now explore connections between critical zone evolution in different parts of the watershed. It is well known that boundary conditions influence the behavior of domains. For example, the behavior of rivers sets the boundary condition for hillslopes, and hence processes that drive fluvial incision ripple throughout the surrounding terrain (e.g., Mudd and Furbish, 2007). We will consider two styles of landscape scale connections where processes or conditions in one area affect the processes or rates of processes in another area. The first of these we have termed downstream mass transfers, in which spatial variation in water runoff and sediment production control river incision, setting the boundary conditions for adjacent slopes. In the second case, which we have labeled upstream-propagating base-level lowering, we describe a cascade of processes that arise from differences in lithology.
5 Downstream mass transfers
The alluvial strath terraces, canyons, and glacial valleys of Boulder Creek (and other Front Range rivers) reflect post-Laramide exhumation of the Front Range (Fig. 4). Evidence of the pre-exhumation topography is suggested by the Ogallala formation, a widespread sandy-gravel unit found along the eastern High Plains, but absent in most areas (including Boulder Creek) immediately adjacent to the mountain front (e.g., Leonard, 2002; Mcmillan et al., 2002). The Ogallala gravels are derived from granites of the Rocky Mountains (Front Range), and therefore indicate that a continuous transport surface must have existed from the range itself across the Plains until the Late Pliocene. In broad brush, therefore, the erosional features illustrated in Fig. 4 represent Latest Pliocene to Quaternary exhumation.
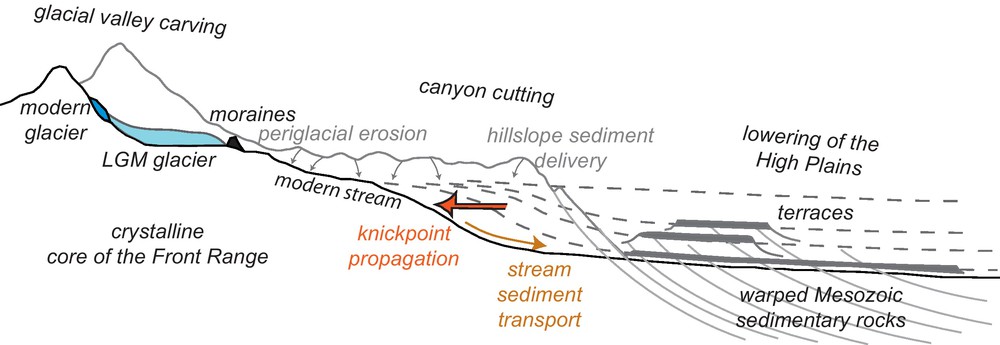
Schematic cross-section of the landscape of the Colorado Front Range illustrating how water and sediment transfers affect erosion in distant regions. Three distinctive erosion regimes exist along the channel: glacial valley carving, canyon cutting, and lowering of the High Plains. These result from the action of glaciers, periglacial and other hillslope sediment transport, and fluvial incision in the streams. Cooler climate at high elevation supports glaciers and periglacial hillslope processes during glacial periods that deliver sediment to the streams (small arrows). Fluvial downcutting occurs during deep interglacials when the sediment supply from upstream declines. Juxtaposition of hard crystalline rock in the Front Range against soft sedimentary rocks of the Plains, result in the most rapid downcutting on the High Plains, leaving a series of alluvial strath terraces. A knickpoint propagates upstream (large arrow) into the hard crystalline rock, carving canyons in the Front Range.
Coupe transversale schématique du paysage dans la Chaîne frontale du Colorado montrant comment les transferts d’eau et de sédiment affectent l’érosion dans des régions distantes. Trois régimes différents d’érosion existent le long du chenal : sculpture d’une vallée glaciaire, incision d’un canyon et abaissement des High Plains. Cela résulte de l’action des glaciers, du transport périglaciaire et autre sédiment de pente et du creusement dans les fleuves. Un climat plus frais à plus haute altitude favorise, pendant les périodes glaciaires, les glaciers et les processus périglaciaires de pente, qui apportent des sédiments aux fleuves (petites flèches). Un creusement vertical fluvial se produit pendant les interglaciaires profonds quand l’apport de sédiment en provenance de l’amont faiblit. La juxtaposition de roches cristallines dures dans la Chaîne frontale et de roches sédimentaires meubles dans les Plains résultent en un creusement vertical plus rapide dans les High Plains, abandonnant une série de terrasses alluviales. Un knick se propage à l’amont (grande flèche) dans la roche cristalline dure, en sculptant des canyons dans la Chaîne.
The key point in this discussion is that prominent landforms within Boulder Creek – the glacial valleys, canyons carved in crystalline rock, and alluvial strath terraces on the Plains (Fig. 4) – can all be plausibly attributed to changes in water and sediment flux from the headwaters of Boulder Creek (Wobus et al., 2010). The connections are hypothesized as follows: Quaternary climate incited glaciation along the crest of the Front Range (Madole et al., 1998), and periglacial conditions over a wider area. Higher sediment flux to rivers during times of glacial climate may derive both from glacier erosion and from sediment export off periglacial hillslopes of the broad, non-glaciated Rocky Mountain surface. Remnants of alluvial fill in Boulder canyon dating to the Last Glacial Maximum (Schildgen et al., 2002) suggest that high erosion and sediment fluxes from the headwaters during glacial times exceeded the carrying capacity of Boulder Creek. On the Plains, recently acquired cosmogenic radionuclide ages of gravels mantling strath terraces reveal alluvial occupation of these surfaces during glacial periods in the Quaternary (Dühnforth et al., 2012). The concurrence of glacial ages for both of these deposits, the alluvial fill stranded on the walls of Boulder canyon and the alluvium on abandoned terraces on the Plains, suggest that high sediment flux from the headwaters during glacial periods both choked the channels within the mountain front, and arrested vertical channel incision on the Plains. A corollary is that during interglacials rivers presumably had excess carrying capacity due to low sediment fluxes, and that this promoted both the erosion of the alluvium from glacial periods and incision into the underlying bedrock. On the Plains, fluvial downcutting of easily eroded sedimentary bedrock during interglacials stranded the broad gravel-capped strath terraces found there (Figs. 4 and 5).
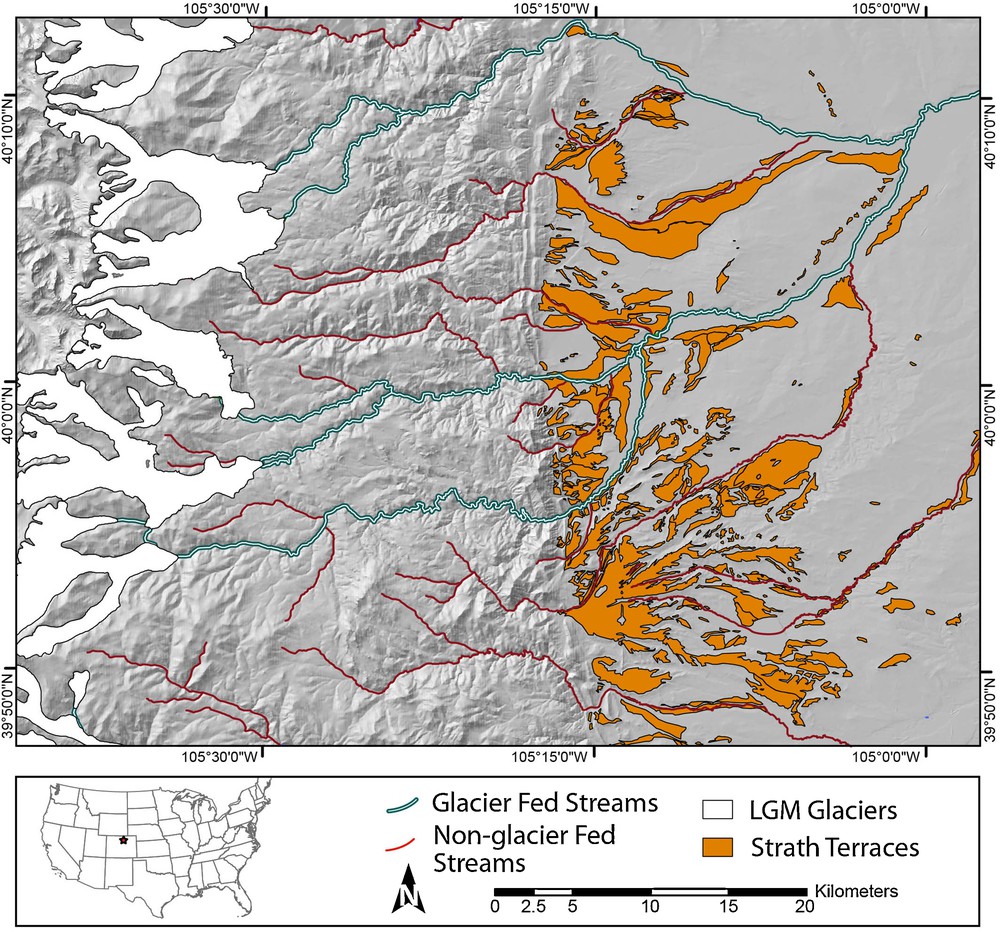
Map of Colorado Front Range and adjacent edge of the High Plains, showing glacier extent at the Last Glacial Maximum (LGM) and the locations of Pleistocene alluvial strath terraces. The strath terraces record exhumation of the Plains as the rivers have cut downwards, and are found lining both glacier-fed and non-glacier-fed rivers. The glacier-fed river systems shown here are South St. Vrain Creek (to the north), and Boulder Creek (to the south), which join on the Plains. Glacier extents from Madole et al. (1998), terraces from Cole and Braddock (2009).
Carte de la Chaîne frontale du Colorado et bord adjacent des High Plains, montrant l’extension du glacier au Dernier Maximum Glaciaire (LGM) et localisation des terrasses alluviales pléistocènes. Les terrasses enregistrent l’exhumation des Plains, quand les rivières les ont érodées verticalement et s’observent à la fois le long des rivières alimentées par le glacier et non alimentées par ce dernier. Les systèmes de rivières alimentées par le glacier ici présentés sont ceux de la South St. Vrain Creek (vers le nord) et de la Boulder Creek (vers le sud), qui se rejoignent dans les Plains. Extension du glacier (selon Madole et al., 1998), terrasses (selon Cole et Braddock, 2009).
Crucial to the argument that the High Plains terraces record climatically driven changes in the critical zone process rates is the notion that rates of sediment delivery to the fluvial system vary strongly with climate, and in particular are high in glacials and low in interglacials. Alluvium-mantled strath terraces are found lining both glacier-fed and non-glacier-fed streams on the Plains (Fig. 5), suggesting that the even non-glacial systems have had climate-modulated sediment fluxes. High sediment fluxes from valleys occupied by glaciers are well known (e.g., Hallet et al., 1996). Periglacial processes are also more active in producing and transporting sediment in the thermal and soil moisture conditions of glacial climates (e.g., Benedict, 1970). We first explored periglacial hillslope models in which lowering of the regolith interface was taken to be a simple function of regolith thickness (Anderson, 2002), and have now extended this approach into models of hillslope fluxes on periglacial landscapes that are modulated by rates of both frost creep of regolith and frost cracking in the subjacent rock (Anderson et al., 2012). It is certainly plausible, if even conservative, that the rates of sediment delivery to the channels in such landscapes vary by factors of more than 2-fold. The variation in sediment supply from the glaciated headwaters of at least some of the major catchments along the front of the Rockies is likely even more pronounced. To first order, the sediment production from a glacierized catchment will depend upon the areal extent of the glacier. In the case of the Front Range, where glaciers completely vanish during interglacials (if the present interglacial is a proper analog of the past), the sediment discharge from these catchments will vary over orders of magnitude. At present, for example, the sediment yields from alpine valleys in the Front Range are small (Pitlick, 1993, and references therein). Debris currently produced from alpine valley walls is trapped as alluvial fans, talus cones and rock glaciers (Leopold et al., 2008), and periglacial features on alpine slopes are less active now than in the past (Benedict, 1970). Little sediment is stored on alpine valley floors. It is therefore likely that the sediment yields from headwater catchments in both glacially and periglacially dominated catchments have varied dramatically from glacial to interglacial climates over the Quaternary.
The toe of the glaciated and periglacial area therefore represents a fulcrum between the locus of sediment production from glacial valleys and periglacial hillslopes and sediment deposition in the fluvial system. The balance shifted from high sediment production in the headwaters during glacial climate to low sediment production in the headwaters during interglacial climate. This climatically driven shift in sediment production in the headwaters led to the opposite response downstream: sediment deposition filling the canyon and mantling the channel in the Plains during glacials, and sediment export and bedrock channel incision during the interglacials. The shift between these states was modulated by varying sediment fluxes from the headwaters, which are themselves controlled by critical zone processes of bedrock weathering and hillslope sediment transport. We note here that in this instance information about climate change, in the form of sediment production (the in Eq. (1)) and sediment discharge (Q in Eq. (1)), is traveling down the landscape: down glacier, down hill, down channel, as depicted in Fig. 4. Hewing of the bedrock by erosion processes was greatest in the headwaters during glacial climates when glaciers and frost cracking processes attacked valleys and hillslopes, but the debris from this chiseling of the high elevations cloaked channels downstream and protected them from incision. This arresting of channel incision downstream probably slowed denudation of the hillslopes adjacent to the alluvium-choked channel. During interglacials, the cessation or reduction in ice-driven erosion in the headwaters allowed the sediment mantle downstream to be swept away. Thus, the warmer climate of interglacials is when the riverbed is exposed to attack, and the sculptor can etch the channel deeper in the downstream parts of the watershed.
6 Upstream-propagating base-level lowering
Since the Late Cenozoic, exhumation of the Mesozoic sedimentary rocks that underlie the western edge of the High Plains has outpaced the erosion of the crystalline rock of the Front Range. This imbalance establishes not only the abrupt topographic break that so markedly delineates the edge of the range (Fig. 1), but also a steep channel gradient that promotes upstream propagation of a fluvial knickzone into the crystalline headwaters (Figs. 2 and 6). Boulder Creek (like other east-flowing rivers in the Front Range) is confined in the mountains to a narrow canyon with steep bedrock-dominated walls from the mountain front upstream to the present position of this knickzone. The upstream-propagating knickzone produces base-level lowering for the slopes and the smaller tributary catchments flanking the canyon. The base-level change incites a cascade of responses, ranging from lowering the watertable to stripping of mobile regolith from slopes. We note that in this instance, geomorphic “information” is being transmitted upstream rather than downstream. That it travels in the opposite direction from that communicated by the downstream motion of sediment serves to knit the landscape together in a yet tighter weave.
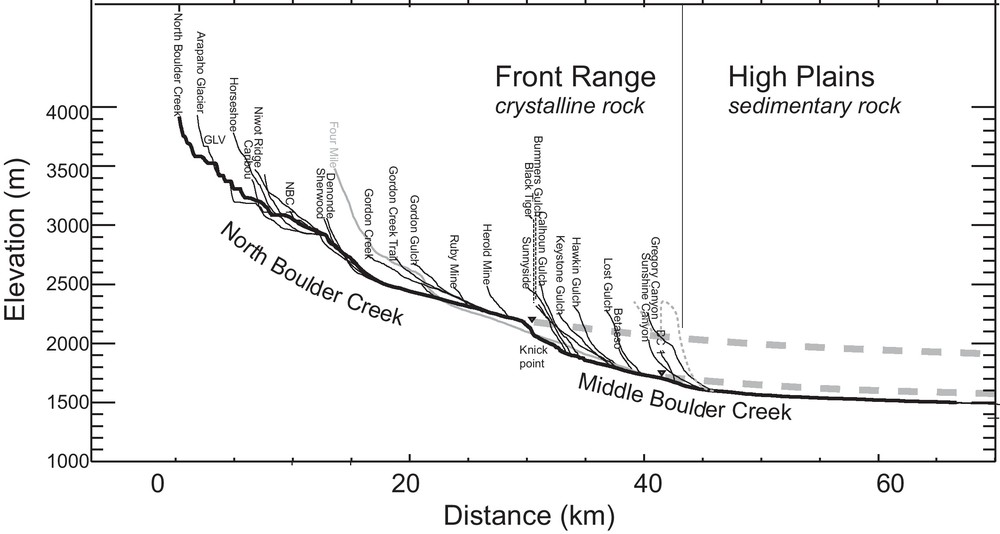
Longitudinal stream profile down North Boulder Creek, which flows into Middle Boulder Creek about half-way down this profile. Thin lines show profiles of tributary streams. A prominent knickpoint (labeled), a few hundred m upstream of the confluence of North and Middle Boulder Creeks, is roughly 12 km upstream from the edge of the mountain front boundary between shale and crystalline bedrock. Note the stepped nature of the profile in the upper few km of the range crest, corresponding to glacial erosion. Two dashed curves are projected from knick zones in the modern profile. The topmost, drawn from the prominent knickpoint on North Boulder Creek, provides a rough estimate of the former position of the profile prior to rapid recent erosion of the High Plains, and suggests a total of roughly 500 m of late Cenozoic incision. The lower curve is projected from a more subtle convexity in the profile, and appears to correspond to one of the prominent terrace levels on the High Plains that have much more recently been abandoned.
Profil longitudinal de fleuve au bas de la North Boulder Creek, qui coule vers la Middle Boulder Creek pour environ la moitié inférieure du profil. Les lignes fines correspondent à des profils d’affluents. Un knick saillant (matérialisé) à quelques centaines de mètres à l’amont de la confluence des North et Middle Boulder Creeks est à peu près à 12 km à l’amont du bord de la limite frontale de la montagne entre roche mère cristalline et roche mère argileuse. À noter aussi la nature en gradins du profil, dans les quelques kilomètres supérieurs de la crête de la montagne, correspondant à l’érosion glaciaire. Deux courbes tiretées sont projetées à partir des zones de knick dans le profil actuel. Celle du haut, tracée à partir du knick sur la North Boulder Creek fournit une estimation grossière de la position initiale du profil avant l’érosion récente rapide des High Plains et suggère une incision totale fini-cénozoïque d’environ 500 m. La courbe du bas est projetée à partir d’une convexité plus subtile dans le profil et apparaît comme correspondant à l’un des niveaux saillants de terrasse des High Plains ayant été abandonné le plus récemment.
The drop of base-level affected by the more rapid incision of the High Plains sedimentary rocks than the crystalline rocks of the range serves as a falling boundary condition for the stream within the range. To first order, this results in a propagating wave of erosion that works headward at a rate governed by the water discharge, and the susceptibility of the rock to erosion. The bedrock river erosion system is well summarized in Whipple (2004), and examples have been worked in many settings. In Boulder Creek, prior work (Anderson et al., 2006) suggests that the celerity of the knickpoint is of order several mm/yr, or several km/Ma, allowing a base-level fall at the range front to be propagated to its present position 12 km from the range front in a few million years. The channel longitudinal profile (Fig. 6) suggests that the lowering of the High Plains has been roughly 500 m over that interval. Boulder Creek is not unique. All major streams draining eastward from the Front Range (and other Laramide ranges of the American West) display profiles with strong convexities at some distance inboard of the mountain front.
Propagation of the knickpoint upstream lowers base-level for the hillslopes lining the river, which then produces a transient response of erosion and sediment flux from the hillslopes (Fig. 7a). The time over which the hillslope sediment flux changes in response to base-level lowering depends on the length of the hillslope, the efficiency of sediment transport, and the ratio of bulk densities of saprolite and mobile regolith (Fernandes and Dietrich, 1997; Mudd and Furbish, 2007). For hillslopes > 100 m in length, like those lining Boulder Creek downstream of the knickpoint, the timescale of response, given typical landscape diffusivities, is likely to be > 106 yrs. Bedrock hillslopes surrounding the knickpoint in Boulder Canyon show that downcutting rates outstrip the rates of mobile regolith generation and rock weathering in this region of most active fluvial downcutting. Downstream of the knickpoint, the canyon widens (Fig. 7a). Hillslope gradients are reduced, but remain dominated by bedrock outcrops. The several Ma since passage of the knickpoint to its present position, more than 10 km upstream from the mountain front, is apparently insufficient to establish a critical zone architecture similar to that found on the Rocky Mountain surface. The continuing evolution of these rock slopes can be considered a response to the climatic events that drove the fluvial incision. Climatic influence on weathering and erosion will be considerably stretched out in time.
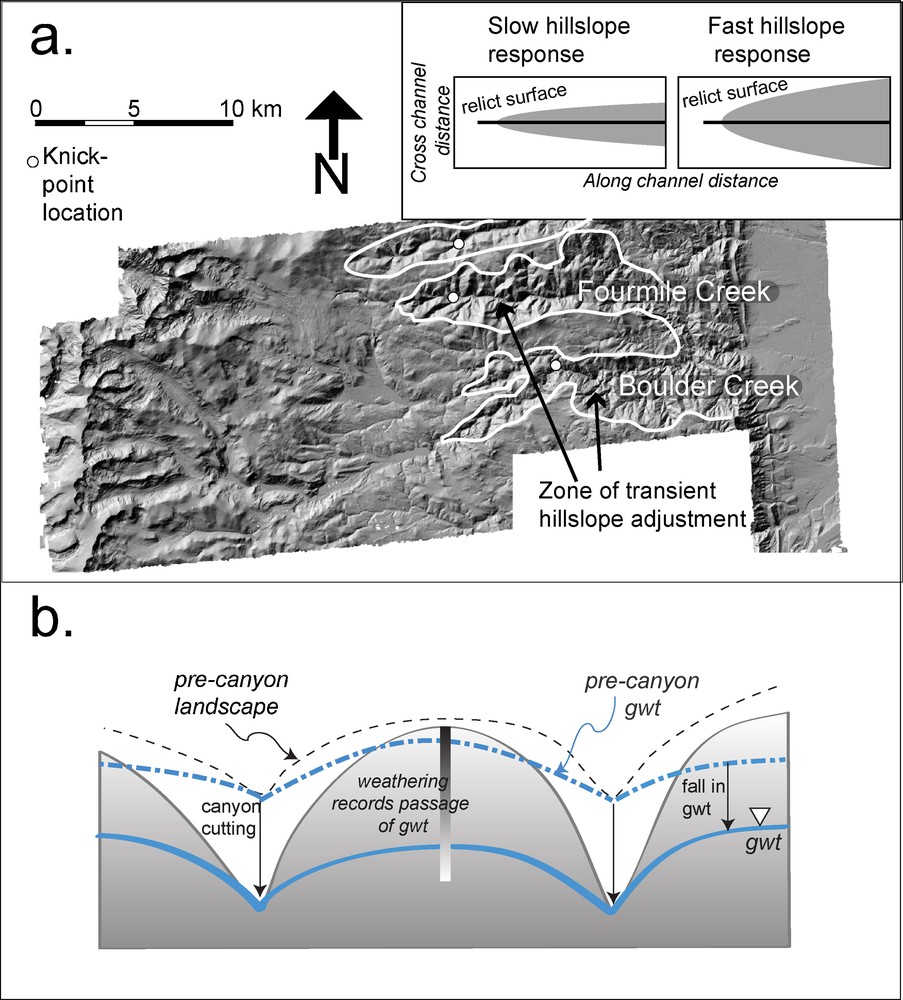
Hillslope and groundwater response to fluvial downcutting. a: LiDAR hillshade of swath of Colorado Front Range, centered on Boulder Creek watershed, outlining (white line) the areas of hillslope adjustment to river incision. The present locations of knick zones in Boulder Creek, a tributary called Fourmile Creek, and a portion of Left Hand Creek (not labeled) are shown with large white dots. Inset shows the relationship between the area of transient hillslope response and knickpoint propagation (or celerity) rates (Mudd and Furbish, 2007). In this LiDAR hillshade, U-shaped glaciated headwater valleys are discernable on western headwaters of the river systems. The low relief Rocky Mountain surface lies between these zones of erosional rejuvenation; b: schematic cross-section across an interfluve of two channels such as Boulder Creek and Fourmile Creek, illustrating the potential influence of an episode of rapid fluvial incision on the groundwater fields in interfluves. Initial topography and associated ground water table (gwt) position shown in dashed lines. Canyon cutting accelerates hillslope response nearest canyons, and a lagged response on the crest. The water table drops as the canyon is cut, increasing the depth of the hydrologic “active layer”. The influence of changes in the groundwater system on weathering is not well known.
Réponse de la pente et de l’eau souterraine à l’érosion verticale fluviale. a : pente ombrée LIDAR du front de la Chaîne du Colorado, centrée sur le bassin versant de la Boulder Creek, soulignant (ligne blanche) les zones d’ajustement de la pente à l’incision de la rivière. La localisation actuelle des zones de knick dans la Boulder Creek, un affluent du nom de Fourmile Creek et une portion de la Left Hand Creek (non matérialisé) sont représentés par de gros points blancs. L’encart montre la relation entre entre la zone de réponse transitoire de la pente et la vitesse de propagation du knick (Mudd et Furbish, 2007). Dans la pente ombrée LIDAR, les vallées d’eaux d’amont modelées en U par les glaciers sont discernables sur les zones d’eaux d’amont occidentales des systèmes de rivières. Le relief bas de la Rocky Mountain Surface se trouve entre ces zones de rajeunissement par l’érosion ; b : coupe schématique au travers d’un interfluve entre deux chenaux tels que la Boulder Creek et la Fourmile Creek, illustrant l’influence potentielle d’une incision fluviale rapide sur les couches d’eau souterraine d’interfluves. La topographie initiale et la position associée de la nappe phréatique (gwt) sont figurées en lignes tiretées. La formation de canyons accélère la réponse de la pente aux canyons les plus proches et une réponse retardée sur la crête. La nappe phréatique chute quand le canyon s’incise, augmentant la profondeur du « feuillet hydrologique actif ». L’influence des changements dans le système d’eau souterraine n’est pas bien connue.
Dropping base-level should also affect the groundwater field, especially when the channel incision is substantial as in the 100s of meters of incision seen in the Front Range (Fig. 7b). Downcutting the channel effectively brings rock into the hydrologic “active layer” – the near surface region of greater permeability due to greater weathering, smaller overburden, and more open fractures (Manning and Caine, 2007). Increased topographic relief increases the proportion of subsurface flow that enters the regional (interbasin) flow system; it also increases groundwater travel times owing to lower potential energy from lowered water table heights (Gleeson and Manning, 2008). The impacts of these changes to the groundwater flow system on chemical processes and rock weathering are not understood at present. While increasing the depth of the hydrologic active layer should enhance rates of weathering of a greater volume of rock, the decline in water flow rates (increased groundwater travel times) could reduce the total solute flux from deep rock weathering.
Surface streams are rare in the terrain lining bedrock canyons in the Front Range. The lack of surface water is a manifestation of lower precipitation in these regions than in the high altitude range crest, but is probably also a legacy of the erosion history. The canyons are not unlike drainage ditches cut into fields. Climate and landscape evolution legacies together shaped the hydrologic system in the Front Range.
7 Conclusions
We have reviewed several cases in which critical zone processes excite a two-way response between upstream and downstream parts of a landscape, using examples from Boulder Creek in the Colorado Front Range. First, we discuss evidence of glacial age alluviation in Boulder canyon and of glacial ages for widespread alluvium mantling strath terraces on the western edge of the High Plains; the ages of these deposits imply that the carrying capacity of channels was exceeded during glacial times. Thus, the downstream regions were sites of sediment deposition, and consequently arrested bedrock erosion, when the headwaters were being carved by the action of glacial and periglacial ice. This is an example of critical zone processes of erosion and sediment production in the headwaters controlling the denudation of downstream portions of the landscape. The climate simultaneously produces high denudation in one area, and deposition and slowed critical zone evolution in another area. Our second example is a teleconnection that migrates upstream. In this case, the happenstance of strong crystalline rocks underlying the upper parts of the watershed and weak sedimentary rocks underlying the lower parts of the watershed has led to uneven exhumation across the watershed as a whole. The greater exhumation of the weak sediments of the High Plains incited generation of a knickpoint in the channel, which migrates upstream, carving canyons into the hard crystalline rocks of the mountain front. This re-excitement of the canyons serves in turn to lower the base-levels of the hillslopes for which the streams serve as boundary conditions. Hillslopes respond slowly to the river downcutting along the canyon, producing a prolonged adjustment of the landscape to the sculptor's blade. Although we have focused more on adjustments in topography and sediment flux, there should also be significant changes in groundwater systems in response to the base-level lowering caused by enhanced channel incision. The lags and system changes that ensue in response to changes in external drivers all confound identification of simple relationships between parameters like chemical weathering flux or soil depth and climate. Landscapes are connected systems in which the movement of sediment and water, and the resulting changes in boundary conditions associated with local deposition or erosion, produce responses that are often out of phase with the drivers. For this reason, the history of the system matters.
Acknowledgements
Two anonymous reviewers provided comments that helped us improve the manuscript. We thank Eric Parrish for help generating Figs. 1, 2 and 5. This work supported by the Boulder Creek Critical Zone Observatory (NSF0724960 and NSF1239281). SPA thanks the French Academy of Sciences, the University of Strasbourg, and François Chabaux for the invitation to participate in the Ebelmen symposium and for supporting her visit to France.