1 Introduction
The majority of the arid to semi arid Moroccan reservoirs are stressed due to enhanced nutrient loading resulting from land runoff and sewage discharges [1–3]. These urban interferences induced eutrophication of the reservoir, while large amounts of the phosphate and nitrogen entering will fuel phytoplankton proliferation [4–8]. In this respect, the Sahela reservoir, constructed in 1994 on the Sahela River, provides drinking water to the entire Moroccan population of Taounate (city located 90 km from Fez, with 1.7 million inhabitants), and has now multiple uses such as hydroelectric production and irrigation. In addition to inputs from the Sahela River, this reservoir receives high amounts of domestic sewage originating from the northeast of the Taounate city through the Guelta El Haila (Sahela River's tributary), which are exacerbated in autumn by the strong non-treated effluents from the proliferating foodstuff processing industry based on olive oil extraction and table olive preparation. Large amounts of the phosphate entering will bring sediments to the hypolimnion and the lake bottom, and undergo exchange between sediments and water under the control of physical, chemical, and biological variables [9–12]. The released phosphate is taken up by microorganisms favoured by the location of phosphatases that may be membrane-bound or confined to the periplasmic space [13–15]. While several authors described seasonal and/or diel changes in the alkaline phosphatase activity from the water column of different ecosystems [8,16–19], none to our knowledge investigated the distribution patterns of the alkaline phosphatase activity (APA) at the water–sediment interface by in situ benthic chamber measurements.
In this paper, we aim to contribute to the understanding of the eutrophication of the reservoir by coupling the exchange of phosphates at the water–sediment interface together with in situ benthic chamber APA and bacteria estimates.
2 Materials and methods
2.1 Study site
The oligo-mesotrophic Sahela reservoir [3], constructed in 1994, has a total volume of . When the reservoir is full, its maximal depth (on dam foundation) reaches 50 m. It is located in the calcareous pre-Rif corridor (about , ) (Morocco) (Figs. 1 and 2). The morphometric characteristics of the reservoir are summarized in Table 1. The water residence time was overall less than one year, ranging between 8 and 11 months. Precipitations averaged 822 mm yr−1, they were irregular (high levels in autumn and winter, but low levels in summer). Water inflow varied from 0.067 Mm3 month−1 in November to 1.66 Mm3 month−1 in March (mean ± sd = 0.79 ± 0.46 Mm3 month−1), with levels decreasing between mid-autumn and spring. Water outflow was null from mid-autumn to spring. From July to October, the water outflow showed a seasonal unimodal distribution, with a peak of 9.96 Mm3 month−1, recorded in September (mean ± sd = 5.41 ± 3.99 Mm3 month−1). Thus, the reservoir was full from March to June and from November to February, whereas from July to October, the hydrological budget was negative (outflows were one fold higher than inflows). Temperature in station 2 varied from 11 °C at 15 m in February to 30.5 °C at 2 m on 25 July (mean ± sd = 19.27 ± 3.38 °C) (Fig. 3). The thermocline established markedly in summer with hot epilimnion (30.5 °C, on 25 July) and warm hypolimnion (16 °C, in 25 July). From December to January, the temperature was homogeneously distributed in the water column. The Sahela reservoir is thus a hot monomictic reservoir. Oxygen concentrations recorded in station 2 varied from 0.64 mg l−1, at 20 m (bottom) on 15 May to 14.4 mg l−1, at 0 m on 28 March (mean ± sd = 5.49 ± 1.59 mg l−1) (Fig. 3). The highest oxygen concentrations were recorded in superficial layers from June to September 1998. Autumnal–spring overturn induced an oxygenation of deep layers with concentrations reaching 4.96 mg l−1 in the bottom on 26 February 1999. Then concentrations decreased as bacteria developed.
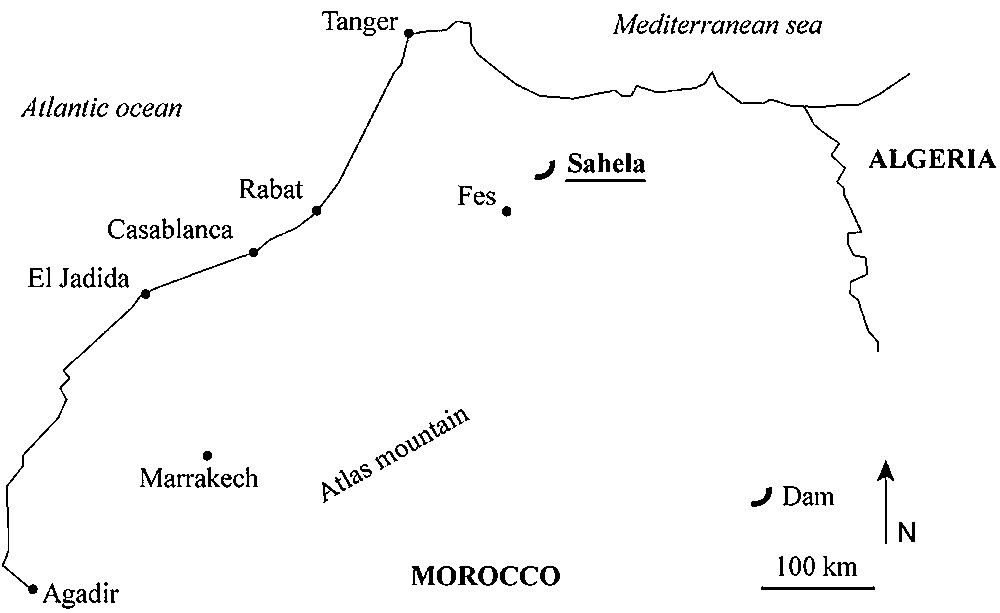
Localization of the Sahela reservoir.

Map showing the two sampling stations.
Morphometric characteristics of the Sahela reservoir
Water filling | 1994 |
Co-ordinates | x=566.8 km |
y=441.4 km | |
Maximum width of the reservoir | 5 km |
Nominal water level elevation | 372 m |
Surface of the catchment | 122 km2 |
Volume | 64.106 m3 |
Surface | 430 ha |
Water residence time | 8 to 11 months |
Minimal depth (station 1) | 13 m |
Maximal depth (station 1) | 18 m |
Minimal depth (station 2) | 15 m |
Maximal depth (station 2) | 22 m |
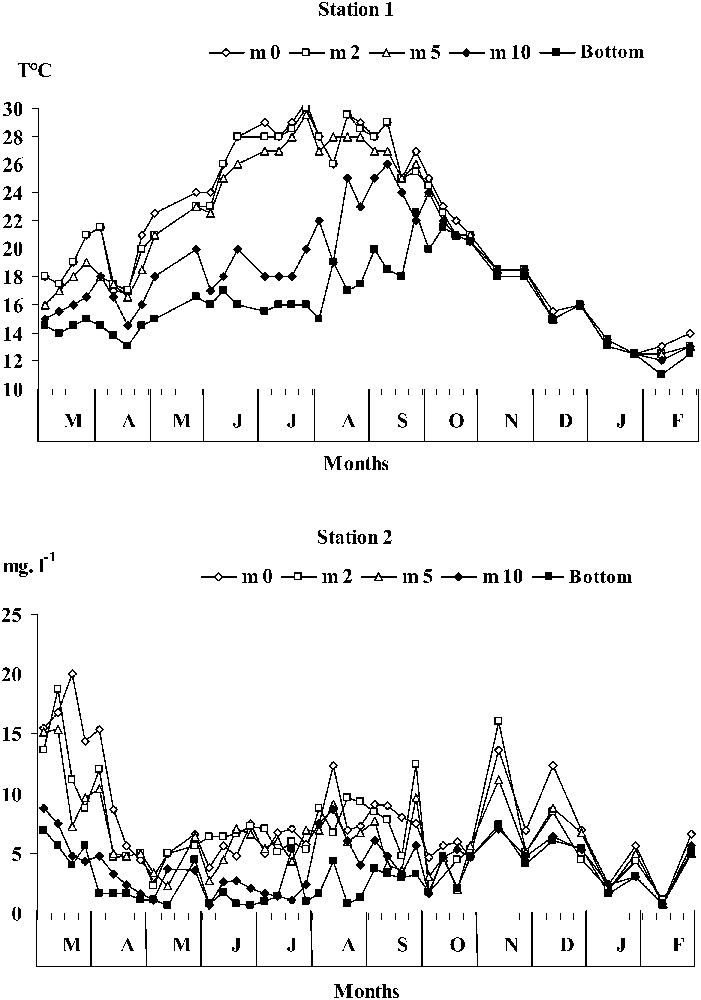
Temporal distribution of temperature (°C) and oxygen concentrations (mg l−1) throughout the monitored water column in station 2.
2.2 Sampling
Two stations were sampled in this study, one near the Guelta El Haila tributary (a branch of the Sahela River) that carries nutrient-rich inputs (including waste water) from the Taounate city (station 1), the second station (station 2) being less influenced by the Taounate interferences (Fig. 2). The release of phosphorus, either real (during the first two days of the experiment) or potential (over the remaining days) at the water–sediment interface was assessed during either low oxic or anoxic in situ conditions with a benthic chamber [1]. Indeed, during our experiments, oxygen concentrations were always <3 mg l−1 (Fig. 3), indicating that the first changes (first slopes) of the environmental factors assessed in this study, such as oxygen concentrations, may be considered as real, since they were similar to those measured outside the benthic chambers. Thereafter, the recorded values reflected only the release potentials, and increased anoxia might be attributed chiefly to containment.
This technique has been reported to reflect correctly the diffusive fluxes that are considered the main upward transport process of phosphates [20]. The chamber used had a surface of 0.4 m2 and a volume of 90 l. It resembles those used in oceanography [21], with two tubes connecting the interior of the chamber to the lake surface where these are both placed on a float. To mimic the in situ hydrodynamic conditions, we used a water mixing system to prevent gradient formation inside the chamber. The chambers used were placed at stations 1 and 2, at 14–15-m and 17–18-m depth, respectively. Samples were taken from the benthic chamber after a preliminary purge of the water contained in the aspirating tube. After each sampling, an equal volume of water was injected into the chamber to avoid bubble formation. Eight samples were taken over 24 days during September at station 1 and 7 over 29 days during October 98 at station 2. In both stations, the volume of water samples ranged between 500 and 1000 ml.
2.3 Chemical elements
Concentrations of orthophosphates and total phosphorus (TP) (after mineralization, which was achieved in an autoclave after H2SO4 attack at 120 °C for 30 min with sodium persulfate) were estimated following Murphy and Riley's technique [22]. We also estimated TP concentrations on prefiltered (at the exit of the pipe, under moderate vacuum, <100 mm Hg) onto GF/C 0.45-mm filter samples. Ferrous iron concentrations were measured after reaction with orthophenantroline and calcium through EDTA complexation. Manganese and aluminium concentrations were estimated by mass spectrophotometry according to NFP 90-048 and NFP 90-0136, respectively.
The fluxes of the phosphorus potentially released (P) expressed in mg m−2 day−1 were calculated from the slope of the curve (P2 – P1/T2 – T1), which reflects the variation with time of P concentrations in the two benthic chambers, each one having a surface on the bottom of 0.4 m2 and a volume of 90 l [1].
Enumeration of bacteria in the benthic chambers was performed by epifluorescence microscopy. Samples were fixed with formaldehyde (2% final concentration) and sub-samples of 3 to 8 ml were stained with acridine orange (0.02%) [23], and filtered on a polycarbonate black filter (Millipore, pore size: 0.2 μm, type GTBP) at low vacuum (<13 kPa). One thousand five hundred to 2000 cells were counted on 20 fields (measurement error not exceeding 7% [24,25]) with an Olympus standard HBS microscope fitted with an HB2-RFL epifluorescence light condenser and a UV source.
The APA (alkaline phosphatase activity) was measured from 100 ml of water sample that were immediately submitted to a differential filtration under moderate vacuum (<100 mm Hg). In order to avoid the average effect resulting from an estimation of bulk APA, and to determine the role of bacterial APA, we fractionated the APA into three size fractions: 0.22–100 μm (total APA), 0.65–100 μm (APA associated with attached bacteria, algae and zooplankton), and 0.22–0.65 μm (APA associated with free bacteria). The filters were placed in sterile tubes supplemented with 3 ml of (Tris-HCl 0.1 M, Mg++ 10−3 M, pH 8.5) buffer and 1 mg ml−1 of p-nitrophenyl phosphate (p-NPP). After incubation during 6 h at 37 °C and under gentle agitation, the enzymatic reaction was stopped by ice cooling the tubes. The spectrophotometric reading was done at 410 nm and the results were expressed in mmol p-nitrophenol (PNP) liberated per litre and per hour for all size fractions. The total specific alkaline phosphatase activity was expressed by the ratio: total APA/total protein concentrations (in μmol PNP liberated per hour). The protein concentrations of the total particulate matter in the benthic chambers (0.22–100-μm size fraction) were determined according to [26] with an albumin (BSA) standard.
3 Results
3.1 Station 1
Oxygen concentrations in the benthic chamber decreased from the first sampling day (0.5 mg l−1) to reach (0 mg l−1) on the 15th sampling day (Fig. 4). The pH also decreased from 8.4 on day 1 to 7.4 on day 22. Total phosphorus concentrations ranged between 0.07 and 0.15 mg l−1 and those of total dissolved phosphorus between 0.02 and 0.1 mg l−1. The distribution of orthophosphate concentrations resembled that of total phosphorus, with levels ranging between 0.02 and 0.05 mg l−1. Peak concentrations for both parameters were recorded on day 22. Calcium concentrations varied from 28 to 34.4 mg l−1. Ferrous iron, manganese, and aluminium were much lower, with levels ranging from 14 to 199 μg l−1, 15 to 21 μg l−1, and 5 to 22 μg l−1, respectively (Fig. 5).

Temporal distribution (from September to October 1998) of pH, dissolved oxygen concentrations (O2), total phosphorus (TP), total dissolved phosphorus (TDP) and orthophosphates , bacterial abundance and alkaline phosphatase (APA) in the two stations of the Sahela reservoir.
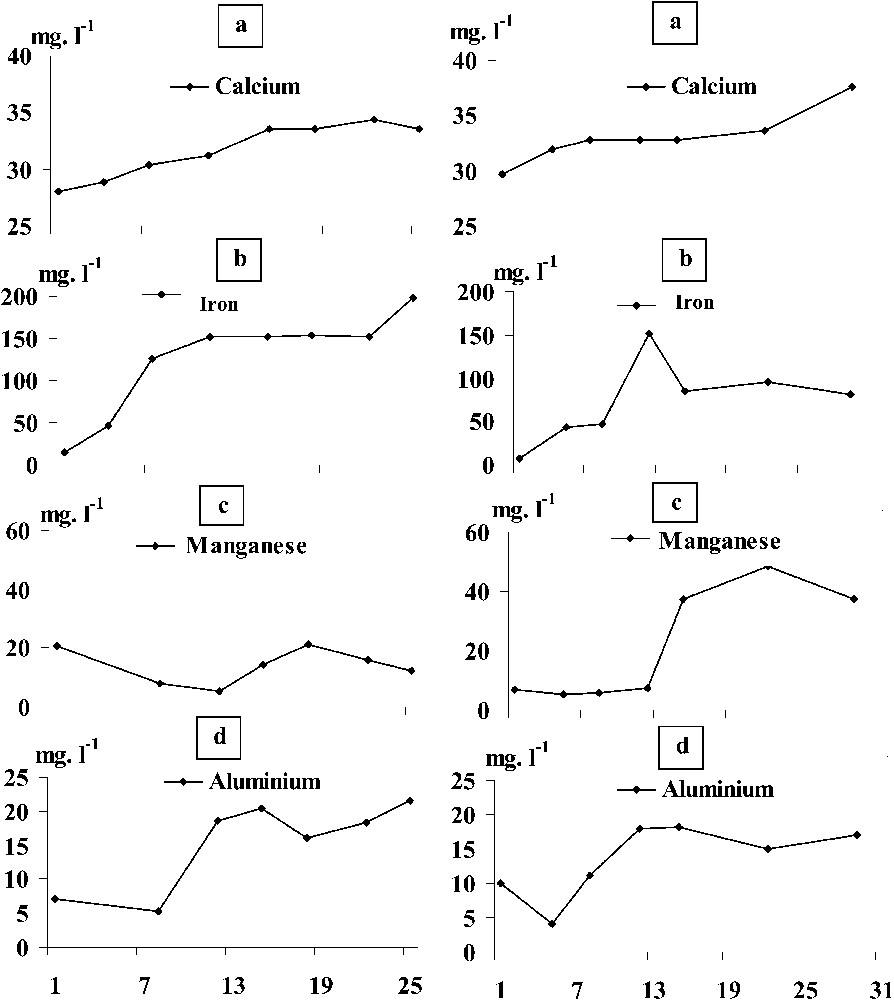
Temporal distribution (from September to October 1998) of calcium (Ca2+), iron (Fe2+), manganese (Mn2+), and aluminium (Al3+) concentrations in the benthic chambers of the two sampled stations.
Bacterial numbers varied from to () (Fig. 4). The temporal distribution of bacterial abundance showed two growth phases. In the first one, bacterial numbers increased from to and then decreased to , the second growth phase was exponential, with cell numbers increasing from to .
The total APA varied from 0.025 to 0.323 mmol PNPl−1 h−1 () (Fig. 4). APA of the 0.22–0.65-μm size fraction ranged between 0.005 and 0.157 mmol PNP l−1 h−1 (), with a relative contribution to the total APA ranging between 20 and 65% (mean 41%). The 0.65–100-μm APA size fraction varied from 0.02 to 0.174 mmol PNP l−1 h−1 (), with a relative contribution to total APA ranging between 35 and 80% (mean 59%). Both the specific APA and APA, being distributed similarly, suggest that phosphatase synthesis was made in the whole pool of proteins. Our assumption may be supported by the highly significant correlation found between both parameters (, ) (Fig. 6).

Temporal distribution of the total alkaline phosphatase activity (APA tot) and its specific activity (APA tot/total proteins concentrations) in the two benthic chambers of the two sampled stations.
3.2 Station 2
Daily fluxes of orthophosphates and total phosphorus (0.85 and 1.35 mg m−2 day−1, respectively) in station 1 were higher than the ones recorded in station 2. In station 2, the daily fluxes of orthophosphates and total phosphorus were higher at the beginning of the study than at the end of the experiments (0.371 and 0.281 mg m−2 day−1, respectively) (Table 2).
Potential fluxes of phosphates released by sediments during either slightly anoxic (i.e. oxygen concentrations < 3 mg l−1) or total anoxic conditions in the Sahela reservoir
Potential fluxes (mg m−2 d−1) | Tot-P | Ca2+ | Fe2+ | Mn2+ | |
Low oxygen concentrations: 0.5–3 mg l−1 | 0.37 | 0.28 | 135 | 2.057 | 0.045 |
Anoxia () | 0 | 0.11 | 128.57 | 5.81 | 2.25 |
Oxygen concentrations in the benthic chamber decreased from day 1 (1.76 mg l−1), but never reached 0 (Fig. 4). The pH decreased from 8 on day 1 to 7 on day 22. Total phosphorus concentrations ranged between 0.03 and 0.05 mg l−1, and those of total dissolved phosphorus between 0.02 and 0.03 mg l−1. Orthophosphate concentrations ranged between 0.02 and 0.03 mg l−1 and they did not change from day 12 to the end of experiments. Calcium concentrations varied from 30 to 37 mg l−1 – this latter value was recorded on day 12. Concentrations in ferrous iron, manganese, and aluminium were much lower than the ones of calcium, with levels ranging from 6 to 151 mg l−1, 5 to 50 mg l−1 and 5 to 18 mg l−1, respectively. The highest concentrations coincided with water anoxia. While calcium and aluminium concentrations were close to those observed in station 1, ferrous iron and manganese concentrations were lower than in station 1 (Fig. 5).
Bacterial numbers varied from to (mean ± sd = 4.83 × 106 ± 1.14 × 106 cells ml−1). The temporal distribution of bacterial numbers in this station resembled that observed in station 1 with, however, higher bacterial densities in station 1 than in station 2 (Fig. 4).
The total APA varied from 0.077 to 0.438 mmol PNPl−1 h−1 (mean ± sd = 0.237 ± 0.155 mmol PNP l−1 h−1) (Fig. 4). 0.22–0.65 μm APA size fraction ranged between 0.018 and 0.131 mmol PNP l−1 h−1 (mean ± sd = 0.052 ± 0.038 mmol PNP l−1 h−1), with a relative contribution to total APA ranging between 14 and 31% (mean 22%). The 0.65–100-μm APA size fraction varied from 0.059 to 0.357 mmol PNP l−1 h−1 (mean ± sd = 0.185 ± 0.113 mmol PNP l−1h−1), with a relative contribution to total APA ranging between 69 and 86% (mean 78%). The temporal distribution of both size fractions was similar to that of total APA. As recorded at station 1, both the specific APA and APA had similar temporal evolutions, suggesting that phosphatase synthesis was made in the whole pool of proteins. Indeed, both parameters were closely related (, ) (Fig. 6).
4 Discussion
The results obtained from the experiments made in the benthic chamber in the two monitoring stations showed that the release of phosphorus was lower than that recorded in the Al Massira reservoir (Morocco) (always higher than 5 mg m−2 day−1 [10]). This may be ascribed to the catchment area's geology, which was marno-calcareous in the Sahela reservoir, but dominated by phosphorus deposits in the Al Massira reservoir, in which a high complexation potential of particulate phosphorus was already reported [1].
Concentrations of Ca2+, Fe2+, Mg2+, and Al3+ measured in the benthic chambers gradually increased throughout the study at stations 1 and 2, most likely due to a solubilization of the particulate matter (Fig. 5). Because enhanced ionic exchanges with the water sediment interface occurred in station 1, higher Fe2+ concentrations were recorded than at station 2. In addition, station 1 is nutrient-enriched by the intense loads or organic matter originating from the urban activities of the Taounate city. Calcium and aluminium concentrations were roughly similar in both stations, most likely due to the catchment geology.
At the first days of the experiments, we measured high iron concentrations, which generated the complexation of orthophosphates resulting from pore water and the dissociation of the low pH-calcico-phosphoric complexes. Similar trends have been reported from different aquatic ecosystems [27]. The decline in orthophosphate concentrations was accompanied by the aerobic bacterial activation together with that of the phosphatase alkaline synthesis, and both algal and zooplankton activities corroborating findings reported in the literature [18,19,28,29]. Moreover, both total APA and its specific activity were lower at station 1 than at station 2, in which we never reached anoxia-inducing release of particulate and thus unavailable orthophosphates. As a result, bacteria underwent a sustained synthesis of alkaline phosphatases [29,30,33]. The examination of the temporal distribution of total phosphorus concentrations confirmed our suggestions, as it clearly showed a higher sediment release of phosphorus in station 1 than in station 2. Size-fractionated (0.65–100 μm) bacteria, being attached to dead organic matter together with algae and zooplankton, were most likely the major contributors (78%) to the total alkaline phosphatase synthesis in both stations (algal contribution to APA was excluded), suggesting that attached bacteria contributed better to APA than free bacteria. This went along with the results reported by [31] from a polluted seaport.
At the two stations, PFe, PMn and PAl complexes dissociated, as from the 7th day onward anoxic conditions prevailed at the bottom. This resulted in high concentrations in calcium, aluminium, and orthophosphates, followed by a steady state (Fig. 5). Concomitantly, anaerobic bacteria proliferated, whereas the APA gradually decreased. This suggests that the synthesis of APA through development of anaerobic bacteria was inhibited as orthophosphate concentrations increased [2,32], which was also confirmed by the decrease of the specific APA (Fig. 6). It seems most likely that anaerobic bacteria did not activate their phosphatases because the latter were depressed by the high orthophosphate concentrations [14]. Furthermore, the temporal distribution of orthophosphate concentrations showed stable and/or low levels at the two stations studied. This pattern may be ascribed to the bacterial assimilation of this element [33,34]. However, the release of phosphorus by sediments may have been favoured by bacteria without involving the APA. Our suggestion went along with the study of several authors [12,18,35] who pointed out the role played by microorganisms in managing phosphorus concentrations in environmental anoxic conditions. At station 1, the bacterial development was sustained by intense loads of urban organic matter (from the Taounate city), resulting in a drastic oxygen depletion at the bottom of the reservoir. Indeed hypolimnetic oxygen concentrations were low from May to August 1998 () due to bacterial degradation of sedimented phytoplankton [36]. These anoxic conditions made the release of phosphorus closely dependent on bacteria, which were shown to solubilize PFe complexes through excretion of low-weight molecules [36,37] and to catalyse iron hydroxide [38,39]. In addition, other works [14,40,41] indicated that the bacterial synthesis of organic acids from the degradation of organic matter yielded a decrease in pH, which induced, when anaerobic conditions prevailed, the dissociation of PCa complexes. Lastly, we should bear in mind that the release of phosphorus is not only governed by the fluctuations of pH and redox potentials [42], but also and strongly by both the extent efficiency of organic matter decomposition in the sediment [43,44]. In the Sahela reservoir, the potential fluxes of phosphate released by sediments were noticeable during either slightly anoxic or total anoxic conditions (Table 2). These levels concomitant with low pH were lower than the ones recorded from previous investigations on the eutrophic reservoir of Grangeant (near Saint-Étienne, France) (38–40 mg orthophosphates m−2 day−1, [6]), and the hypereutrophic reservoir of Villerest (near Roanne, France) (114.8 mg orthophosphate m−2 day−1, [1]). In Lake Aydat (France), the release of orthophosphates reached 40 mg m−2 day−1, and was also induced by low pH and anoxia [45].
5 Conclusion
Phosphorus exchange within the sediment–water interface is determined by the combined effects of the physical-chemical variability of the environment, the geological composition of the catchment basin, and the alkaline phosphatase activity of bacteria. The dissociation of calcium-bound phosphorus was chiefly governed by the pH decrease, whereas that of iron-bound, aluminium-bound, and manganese-bound phosphorus was linked to anoxia. The latter induced enhanced heterotrophic degradation of organic matter by proliferating bacteria, resulting in the release of phosphorus and ammonium that depleted oxygen and decreased pH. In the absence of a marked thermal stratification in the Sahela reservoir, and because of sustained strong winds recorded in this area, it is probable that a fraction of the released phosphate would diffuse upwards to the euphotic zone initiated by advective, dispersive, and convective water movements. This phosphorus added to that brought by terrestrial runoff (low-flow conditions) from upstream will promote rapid algal growth, due to high water residence times, abundant irradiances, and high water temperatures [46–49]. At present, the Sahela reservoir is starting to experience poor water quality and the persisting discharge of organic matter, sewage, olive mill waste will increase these problems. To improve our understanding of the progressive eutrophication of the Sahela reservoir, we are currently investigating the impact of internal phosphate dynamics on the seasonal development of the phytoplankton community. Our study may help to plan the best disposal options for anthropogenic wastes.
Acknowledgements
We gratefully acknowledge support from the staff of the Sahela reservoir. We thank one anonymous referee for helpful advices and comments. This work was supported by a French–North African programme aiming at studying the determinism of eutrophication in developing countries.