Abreviations
VLCFAs
very long chain fatty acids
KCS3-keto-acyl-CoA synthase
KCR3-keto-acyl-CoA reductase
HCD3-hydoxy-acyl-CoA dehydratase
ECRtrans 2,3-enoyl-CoA reductase
TAGtriacylglycerol
PCDprogrammed cell death
LCBlong chain base
DHSdihydrosphingosine
3-KDHS3-ketodihydrosphingosine
PEphosphatidylethanolamine
PCphophatidylcholine
PSphosphatidylserine
PGphosphatidylglycerol
MGDGmonogalactosyl-diacylglycerol
DGDGdigalactosyl-diacylglycerol
1 VLCFAs: introduction
Very long chain fatty acids (VLCFAs) are fatty acids with an acyl chain of 18 carbons and longer. The chain length, the degree of unsaturation, the type of polar head and the associated lipids provide the structural and functional diversity of these fatty acids. In plants, VLCFAs are found in triacylglycerols as seed storage lipids. They are involved as membrane constituents and signalling molecules in sphingolipids and phospholipids. Finally, VLCFAs are necessary for the production of cuticular waxes and suberin, two extracellular biopolymers preventing plants from desiccation or external aggressions.
VLCFAs are involved in similar functions in animals, i.e. storage lipids in adipose tissues, the protective lipid barrier of the skin and membrane structural components. Genetic studies on VLCFAs synthesis during the last 10 years are now providing new insights on the cellular functions of VLCFAs. In this review, we will mainly focus on the involvement of VLCFAs in plant development.
2 Biosynthetic pathway and enzymes
VLCFAs are synthesized by the sequential addition of two carbons through four successive enzymatic reactions gathered in the endoplasmic reticulum within a protein complex named the elongase (Fig. 1). The first step of fatty elongation is the condensation of a long chain acyl-CoA with a malonyl-CoA by the 3-keto-acyl-CoA synthase (KCS or condensing enzymes). The resulting 3-keto-acyl-CoA is then reduced by a 3-keto-acyl-CoA reductase (KCR) generating a 3-hydroxy-acyl-CoA. The third step is the dehydration of the 3-hydroxy-acyl-CoA by a 3-hydoxy-acyl-CoA dehydratase (HCD) to a trans-2,3-enoyl-CoA, which is finally reduced by the trans 2,3-enoyl-CoA reductase (ECR) to yield a two-carbon elongated acyl-CoA. Once acyl-CoA has been elongated from the elongase complex, they can be incorporated into different lipid classes.
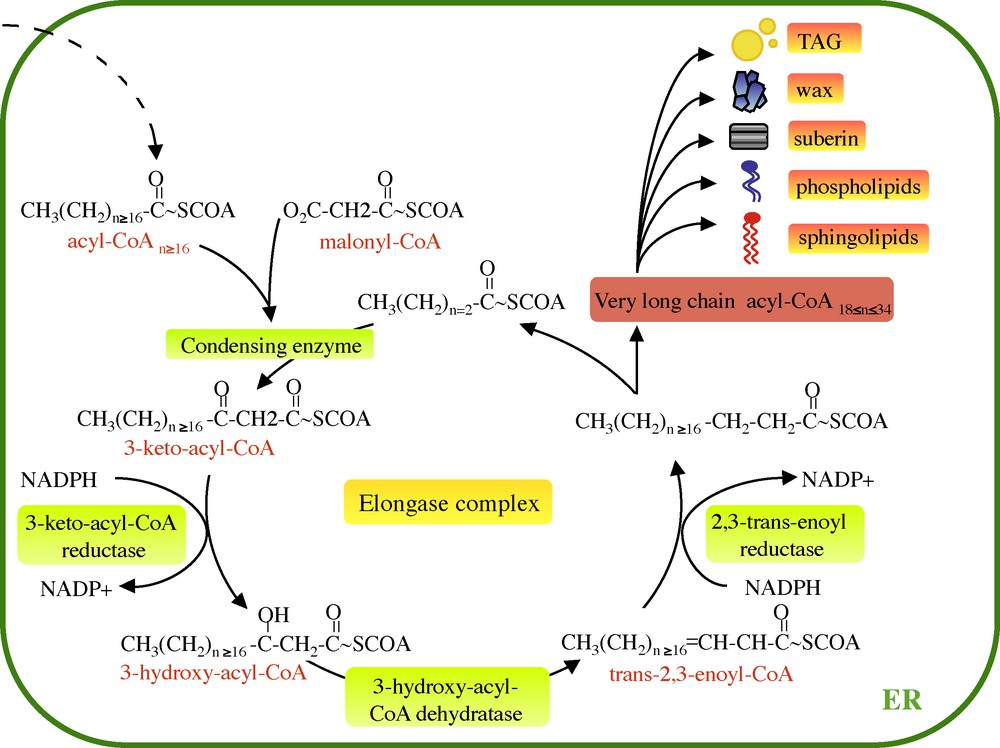
Very long fatty acid elongation cycle. VLCFAs are elongated from long chain acyl-CoA (n ≥ 16) by the ER-associated elongase complex. Each two carbon cycle requires four successive enzymatic steps, the condensation of the malonyl-CoA with the acyl-CoA, reduction of the 3-keto-acyl-CoA, dehydratation of the 3-hydroxyacyl-CoA and the reduction of the trans-2,3-acyl-CoA. Finally, the enoyl-CoA gives rise to the formation of very long chain acyl-CoA. ER: enplasmic reticulum.
VLCFAs are usually elongated from pre-existing C18 acyl-CoAs produced by the cytosolic fatty acid synthase complex (FAS). However, it has been recently demonstrated that C16-CoA could be also a substrate for the KCS since a mutation in the dehydratase gene led to the accumulation of 3-hydroxyC18-CoA [1].
In yeast and mammals, the KCS proteins are encoded by the multigenic ELO gene family. These enzymes were found to have different substrate specificity that depends on their chain length and their degree of unsaturation [2]. For instance in yeast, Elop2 is responsible for the elongation of VLCFAs up to c24, while Elop3 is crucial for the conversion of C24 to C26 [3]. In Arabidopsis genome, KCS enzymes are encoded by an ELO-like family of four putative condensing enzymes and 21 FAE1-like KCS genes [4,5] (Table 1). Although FAE1-like enzymes are structurally unrelated to the ELO enzymes, it has been shown that several FAE1-like KCS can rescue lethality of the yeast Δelo2/Δelo3 double mutant [2]. Two condensing enzymes were characterized in details: FATTY ACID ELONGATION 1 (FAE1), which encodes a condensing enzyme responsible for the C20 and C22 fatty acid elongation involved in seed triacylglycerol [6] and CER6, which catalyzes the elongation of fatty acids longer than C22 specifically in epidermal cells [7,8]. The KCS CER6 [7], KCS1 [9] and FIDDLEHEAD (FDH) [10,11] were found to be involved in the synthesis of VLCFAs precursors of shoot waxes: a complete loss of function of CER6 and KCS1 resulted in accumulation of C24 wax derivatives suggesting a potential role of these enzymes in C24 elongation. Similarly, fdh mutant presented elevated levels of C20:0 and C22:0 fatty acids in fdh-derived cell wall material [12]. Recently, two redundant KCS, KCS20 and KCS2/DAISY, have been shown to be necessary for C20 to C22 elongation in the biosynthesis of cuticular waxes and root suberin [13]. In conclusion, the KCS multigene family is associated with substrate specificity mainly on the basis of chain length (C20–C22 vs C26+ for instance), but also with the nature of the downstream VLCFAs lipid pool that depends on the tissue-expression pattern (FAE1 and triacylglycerols vs DAISY and suberin).
Arabidopsis elongase genes.
Gene names | Others names | Arabidopsis accessions | Yeast homologs | Biochemical functiona | Activityb | Phenotypec |
KCS1 | KCS1 | At1g01120 | – | 3-keto-acyl-CoA synthase Wax/suberin biosynthesis (c26) [9] |
[50,51] | Growth retardation [9] |
KCS2 | DAISY | At1g04220 | – | 3-keto-acyl-CoA synthase | [8,51] | Glossy appearance [13] |
Wax/suberin biosynthesis (c22) [13] | Root growth retardation/Suberin layer alteration | |||||
KCS3 | – | At1g07720 | – | Putative 3-keto-acyl-CoA synthase | – | – |
KCS4 | – | At1g19440 | – | Putative 3-keto-acyl-CoA synthase | – | – |
KCS5 | CER60 | At1g25450 | – | Putative 3-keto-acyl-CoA synthase | [51] | – |
KCS6 | CER6, CUT1 | At1g68530 | – | 3-keto-acyl-CoA synthase | – | Glossy appearance/ Reduced fertility |
Wax/suberin biosynthesis [7,52,53] | [7,51,53] | |||||
KCS7 | – | At1g71160 | – | Putative 3-keto-acyl-CoA synthase | – | – |
KCS8 | – | At2g15090 | – | Putative 3-keto-acyl-CoA synthase | – | – |
KCS9 | – | At2g16280 | – | Putative 3-keto-acyl-CoA synthase | [8] | – |
KCS10 | FDH | At2g26250 | – | Putative 3-keto-acyl-CoA synthase | – | Postgenital organ fusions/Reduced fertility |
Trichome defects [10,12] | ||||||
KCS11 | – | At2g26640 | – | Putative 3-keto-acyl-CoA synthase | – | – |
KCS12 | – | At2g28630 | – | Putative 3-keto-acyl-CoA synthase | – | – |
KCS13 | HIC | At2g46720 | – | Putative 3-keto-acyl-CoA synthase | – | Stomatal density augmentation in response to elevated CO2 [54] |
KCS14 | – | At3g10280 | – | Putative 3-keto-acyl-CoA synthase | – | – |
KCS15 | – | At3g52160 | – | Putative 3-keto-acyl-CoA synthase | – | – |
KCS16 | – | At4g34250 | – | Putative 3-keto-acyl-CoA synthase | – | – |
KCS17 | KCS2 | At4g34510 | – | Putative 3-keto-acyl-CoA synthase | [50,51] | – |
KCS18 | FAE1 | At4g34520 | – | 3-keto-acyl-CoA synthase | [2,8,50,55] | |
Seed storage lipids (c20/c22) [6] | ||||||
KCS19 | – | At5g04530 | – | Putative 3-keto-acyl-CoA synthase | – | – |
KCS20 | – | At5g43760 | – | 3-keto-acyl-CoA synthase | [8,51] | Glossy appearance [13] |
Wax/suberin biosynthesis (c22) [13] | Root growth retardation/Suberin layer alteration | |||||
KCS21 | – | At5g49070 | – | Putative 3-keto-acyl-CoA synthase | – | – |
Elop-like | FEN1 | At3g06460 | ELO2 | Unknown | – | – |
Elop-like | FEN1 | At3g06470 | ELO2 | Unknown | – | – |
Elop-like | GNS1/SUR4 | At1g75000 | ELO3 | Unknown | – | – |
Elop-like | GNS1/SUR4 | At4g36830 | ELO3 | Unknown | – | ABA hypersensitivity (reduced root growth inhibition reduced germination) reduced water loos from leaves [56] |
KCR1 | KCR1 | At1g67730 | YBR159w | 3-keto-acyl-CoA reductase | – | Embryo lethality (null allele) |
General reduction VLCFA content [15] | Glossy appearance/postgenital organ fusions Trichome defects Lack of lateral roots/reduced hair roots [15] |
|||||
KCR2 | KCR2 | At1g24470 | YBR159w | Unknown | – | – |
HCD | PAS2 | At5g10480 | PHS1 | 3-hydroxy-acyl-CoA dehydratase | – | Embryo lethality (null allele) |
General reduction VLCFA content | Glossy appearance/Postgenital organ fusions | |||||
3-hydroxy-acyl-CoA increase [1] | Cell proliferation defects [1] | |||||
HCD2 | PAS2-like | AT5G59770 | PHS1 | Unknown | – | – |
ECR | ECR10 | At3g55360 | TCS13 | Trans-2,3-enoyl-COA reductase | – | Glossy appearance/Postgenital organ fusions/Reduced fertility |
General reduction VLCFA content [17] | Trichome defects Cell expansion defects [17] |
a Biochemical function is indicated (with acyl-chain specifity).
b Activity: when enzymatically active KCS is demonstrated a reference is provided.
c Phenotype of mutant or RNAi lines.
The 3-ketoacyl-reductase protein is encoded by a single gene in yeast named YBR159w and by two genes in maize GL8A and GL8B. The gl8agl8b double mutant was found to be embryo lethal, demonstrating the essential role of VLCFAs in plant development. Analysis of single mutants showed that KCR activity is required for cuticular wax production [14]. In Arabidopsis, two KCR homologs, KCR1 and KCR2, have also been identified, but only KCR1 has been demonstrated to be involved in the fatty acyl elongase complex since it complemented the ybr159w yeast mutant. kcr1 null alleles are also embryo lethal in Arabidopsis [15].
The dehydratase gene was only discovered recently in yeast as the YJL097w/PHS1 gene [16]. This gene is essential for cell viability mainly during cell division since weak phs1 allele showed G2/M transition defects. Like for KCR, Arabidopsis genome contains two PHS1 homologs, PASTICCINO2 (PAS2) and At5g59770. PHS1 expression complements pas2-1 defects, however, only PAS2 was able to rescue phs1 lethality [1] (Fig. 2A). A complete loss of PAS2 activity led also to embryo lethality stressing the essential role of VLCFAs (Fig. 2B). Partial loss of PAS2 function led to the general reduction of VLCFAs in triacylglycerols, complex sphingolipids and cuticular waxes while it accumulates long chain bases and precursors of sphingolipids. Biochemical phenotype was associated with strong developmental defects. Surprisingly, whereas KCS enzyme was thought to be the only limiting step for VLCFAs elongation, the overexpression of dehydratase activity in Arabidopsis led to enhanced VLCFAs mainly in epicuticular waxes suggesting that several limiting enzymatic steps were present among the elongase complex [1].
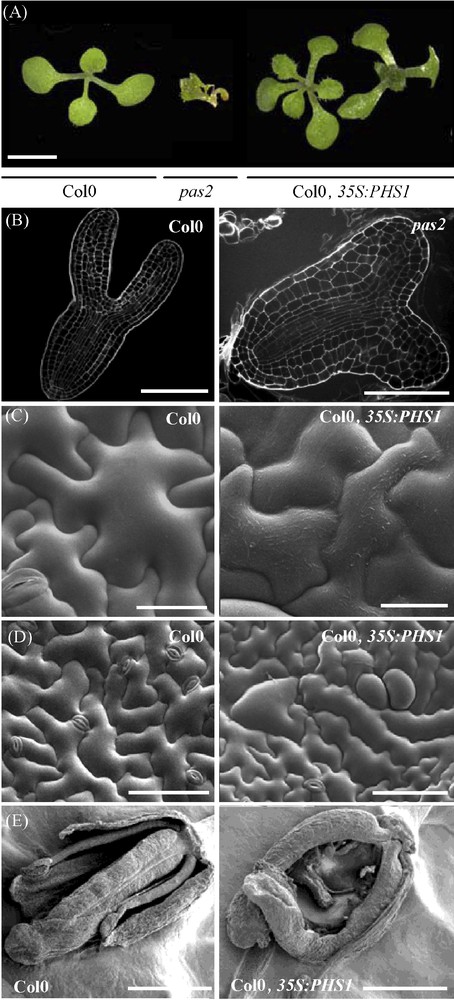
PAS2 is essential for plant development. A. pas2 mutation and expression of 35S:PHS1 lead to abnormal leaf morphology (fusions, left) and phyllotaxy (triple cotyledons, right) (scale bar, 1 mm). B. The pas2 embryo (right) at the torpedo stage showed defective cotyledon development compared to wild-type (left) (scale bar, 40 μm). Embryos were fixed and stained with propidium iodide and imaged with a scanning confocal microscope. C. Scanning electron microscopy (SEM) showed increase of epicuticular waxes in PHS1 expressing epidermal cells (right) compared to wild-type (left) (scale bar, 100 μm). D. PHS1-expressing plants have an increased wax load on epidermal cell surface (right) compared to wild type (left) (scale bar 50 μm). E. PHS1-expressing plants showed unfused carpels (right) compared to wild type (left) (scale bar, 1 mm). Masquer
PAS2 is essential for plant development. A. pas2 mutation and expression of 35S:PHS1 lead to abnormal leaf morphology (fusions, left) and phyllotaxy (triple cotyledons, right) (scale bar, 1 mm). B. The pas2 embryo (right) at the torpedo stage showed ... Lire la suite
The last reduction step in the elongase complex is carried out by the trans-2,3-enoyl-reductase TSC13 gene in yeast and was so far associated with only one gene in Arabidopsis named CER10 [17]. Like PAS2, the absence of ECR activity resulted in general reduction of VLCFAs. Even though CER10 is the only ortholog of the yeast TCR13 ECR, this gene is not essential unlike KCR1 or PAS2, indicating the potential existence of functionally equivalent isoforms of ECR in Arabidopsis [15].
Once elongated, the very long chain acyl-CoA is incorporated into various lipid pools comprising triacylglycerols, epicuticular waxes, phospholipids and sphingolipids. The relative roles of these different pools for plant growth and development are variable, but recent contributions highlight the importance of VLCFAs and the potential role of fatty acyl chain length in plant development (Table 1).
3 TAG are not involved in plant development
The triacylglycerols (TAG) are formed by the Kennedy pathway from a single molecule of glycerol, in which each hydroxy group is acylated with up to three acyl chains ranging from eight to 26 carbons long. They are stored in oleosomes during the embryo or/and endosperm maturation. These lipids act as a reserve of energy for germinating seeds and they can represent about half of the total seed dry weight. In the majority of seeds, acyl groups from TAG are similar to those found in membrane lipids. For instance, the most abundant fatty acids in seeds are palmitic acid (16:0), stearic acid (18:1), oleic acid (18:0), linoleic acid (18:2) and linolenic acid (18:3). However, VLCFAs can be also present in TAG in significant amount. Members of Brassicaceae family for instance preferentially incorporate fatty acids from mono-insaturated VLCFAs. Millar et al. have determined the seed oil fatty acyl composition for 100 different Arabidopsis accessions [18]. They showed that all Arabidopsis accessions contained a significant proportion of VLCFAs averaging 31% w/w of total seed fatty acid. The most abundant and specific VLCFAs in TAG are the ecosenoic acid C20:1 (20% of the total seed fatty acid) and the erucic acid C22:1 (2% of the total seed fatty acid).
To date, no real physiological or biochemical role of VLCFAs in TAG composition has been demonstrated. The fae1 mutant, which contains less that 1% w/w of VLCFAs [6], is still able to germinate. Interestingly, even if FAE1 gene expression is restricted to the seed, fae1 mutations modified vegetative development with early flowering transition and slower leaf growth [19]. Similarly, Canola rapeseed varieties are completely devoid in VLCFAs in their seed oil composition and do not show significative modification in terms of growth. Thus, these results suggest that the VLCFAs in TAG are not essential for plant development.
In animals, VLCFAs are also used to generate energy since they can be oxidized by beta-oxidation in peroxisomes and by the cytochrome P450 CYP4A. Several studies have demonstrated that an accumulation of VLCFAs in the liver resulting from a disrupted VLCFAs oxidation can be an important cause of disease [20]. Deficient mice in peroxisomal fatty acyl-CoA oxidase (AOX) present hepatic steatosis and steatohepatitis.
4 VLCFAs in extracellular biopolymers and development
The cuticle forms a hydrophobic barrier, which covers the aerial parts of land plants. The cuticle consists of a polyester matrix of C16 or C18 hydroxy and epoxy-hydroxy-fatty acids named cutin embedded and overlaid by cuticular waxes. These waxes are made by a mixture of very long chain lipids of 26 to 34 carbons long such as primary and secondary alcohols, aldehydes, alkanes, ketones and esters.
Elongated acyl-CoA are substrates of two distinct biosynthetic pathways leading to wax synthesis: the decarbonylation pathway and the reduction pathway. The decarbonylation pathway begins with the production of aldehydes from acyl-CoA by the acyl-CoA reductase. Then, aldehydes are decarbonylated by an aldehyde decarbonylase to produce alkanes with odd-chains. These alkanes are then hydroxylated to form secondary alcohols, which are finally oxidized in ketones [21]. The reduction pathway is used for primary alcohols and waxes ester synthesis. Several reports showed that primary alcohols are directly synthesized from acyl-CoA by an acyl-CoA reductase. These primary alcohols can then be combined with free fatty acids to form wax esters by an acyl-CoA alcohol transferase.
Specific acyl chain length predominates in each lipid class conferring different physical/chemical properties to waxes, in particular modifying their form and appearance. For instance, Arabidopsis stems show a crystalline wax structure whereas leaves have a thin wax layer. There is also a report suggesting that similar crystalline wax structure may originate from different lipid compositions [22].
The proportion of the major classes differs among plant species, and also among tissues and organs. For instance, alkanes and ketones are mainly found in leek leaves and in Arabidopsis stems, whereas they are undetectable in maize leaves. Specific differences can even be found between the adaxial and abaxial leaf surface of Pisum sativa that show 10-fold difference in the levels of alkanes [23]. Finally, wax composition depends on development stages. Young maize leaves have 63% of primary alcohols whereas old leaves have 42% of predominantly wax esters [24].
In many plants, cuticular waxes confer a glaucous or grayish appearance on the plant surface. This phenotype led to a powerful genetic screen that allowed the isolation of the eciferum mutants (cer) characterized by reduced epicuticular waxes. Several mutants turned out to be mutations in elongase genes. Indeed, cer6 mutant abolished wax production on Arabidopsis stems [7] and cer10 mutant showed 60% reduction of wax load [17]. Although the rest of the elongase mutants have not been identified as cer mutants, they also exhibit a waxless phenotype correlated with reduced wax loads. The kcs1 mutant presents 80% less aldehydes and alcohols of C26 and C30 length on leaves, stem and siliques [9]. In pas2 plants, all the wax aliphatic components are strongly reduced [1]. Finally, the AtKCR1-RNAi lines, which are defective in the keto-acyl-CoA reductase activity, present a glossy aspect and a reduced cuticular wax load [15].
Interestingly, the core elongase mutants (pas2, kcr1, cer10) display another obvious phenotype, which consist in postgenital organ fusions. Usually, such fusions are observed in angiosperm during the formation of the carpel. Studies in Catharanthus roseus suggested that organ fusion is caused by specific epidermal cell interactions involving exchange of small water-soluble morphogenetic factors [25]. Several Arabidopsis mutants showing organ fusion were characterized by a permeability change of the outer epidermal cell wall and cuticle [12]. As the epidermal layer is considered as an active site of lipid synthesis necessary for deposition of cuticle and waxes, it has been postulated that alteration of the cell wall or the cuticle could enhance the diffusion of fusion promoting signals. Conversely, the overexpression of PHS1 led to an increased wax load on the epidermal surface of leaves, stems and carpels (Fig. 2C). Leaves were smaller, more digitated and showed abnormal cell shape [1]. Carpels were unfused demonstrating that epicuticular waxes at the epidermal surface are essential to prevent organ fusion (Fig. 2D and E).
Mutants with reduced waxes do not always present organ fusion. For instance, the most dramatical reduction in wax load was observed in CER6-silenced plants (7% of that of the WT) without any consequence on growth or on plant development [7]. Mutation in FDH, which has also been implicated in the synthesis of VLCFAs precursors for wax production [12], leads to ectopic fusion phenotype but without glossy leaves or stems that are typical of cer phenotype [10].
The fact that cer6 mutant is missing specifically acyl-CoA C26 or longer suggests that at least a specific acyl chain length might be necessary for preventing postgenital organ fusion. Thus, examining the involvement of each component of the cuticle and epicuticular waxes will be necessary to understand how epidermal fusion is initiated.
VLCFAs are also major components of another extracellular biopolymer, the suberin that can be found in diverse tissues but mainly in the root endodermal cells as characteristic lamella structure that contains aliphatic chains and phenolic components. The main aliphatic monomers are glycerol associated with ω-hydroxyacids (ω-OH-acids), and α,ω-dicarboxilic acids (α,ω-diacids), ranging from C16 to C30 in chain length. Unsubstitued fatty acids, primary alcohols and an aromatic domain rich in ferulic acid are also commonly found in suberin.
Suberin biosynthesis begins in the symplast where its building blocks are found. Depending on the species, the fatty acids can undergo mid-chain modification leading to unsaturated and to epoxy-fatty acids, whereas saturated fatty acids are elongated up to C30 by the elongase complex. The α,ω-diacids and ω-hydroxyacids are produced by ω-oxidation catalized by a NADPH dependent cytochrome P450 mono-oxygenase. After export to the apoplast, the monomers are extensively polymerized in suberin macromolecule.
In Arabidopsis roots, free fatty acids are mainly C22:0 while primary alcohols includes 18:0, 20:0 and 22:0. However, VLCFAs are less abundant in ω-OH-acids (mainly 18:1 but 22:0 and 24:0 species can be detected) and absent in α,ω-diacids (only 18:1 and 16:0) [26].
Little is known on the role of the suberin during plant development. Recent studies have demonstrated that a modification of suberin structure or composition could alter root development. The double mutant kcs20/daisy-1 that showed specific reduction of C20+ acyl chains in suberin exhibited reduced root growth indicating that specific VLCFAs in suberin are required for root development [12]. The kcr1 RNAi lines present also an abnormal root phenotype, with an inhibition of lateral root elongation and a strong reduction of root hair length that are associated with reduced levels of C20+ in root fatty acids. Three novel peaks in suberin composition corresponding to C16 and C18 α,ω-diacids and C18 ω-hydroxy fatty acids were also observed [15].
Like plant epidermal waxes, animal skin is coated with a protective hydrophobic barrier against external aggression. Interestingly, the mammalian ELOVL3 condensing enzyme has been suggested to be involved in the formation of fatty acyl chains containing up to 24 carbons required in the epidermis for hair and skin formation. Indeed, Elovl3 ablated mice exhibited a sparse hair coat and a general hyperplasia of the sebaceous glands that led to loss of the protective function of the skin. These phenotypes correlated with significant modifications of the lipid content of the epidermis, which is a mixture of triglycerides, wax and sterol esters. More precisely, the elovl3 mice displayed high levels of eicosenoic acid (20:1), which was more accentuated in triglycerides fractions. Thus, VLCFAs, by being components of protective extracellular biopolymers, are essential during development in both plants and vertebrates.
5 VLCFAs in membrane lipids: signal molecules and membrane structural components
Biological membranes consist in a bilayer of polar lipids, predominantly glycerolipids associated with sterols and sphingolipids. In plant membranes, two distinct glycerolipid groups are found: the phospholipids, which contain a phosphate as polar head group, and the glycolipids, harbouring a carbohydrate moities on their head group. Among plant phospholipids, phosphatidylethanolamine (PE) and phophatidylcholine (PC) are the major components of the plasma membrane, mitochondrial membrane and endoplasmic reticulum, whereas phosphatidylserine (PS) is a minor lipid accounting for less than 1% of the total glycerolipids. On the other hand, glycolipids such as monogalactosyl-diacylglycerol MGDG and digalactosyl-diacylglycerol DGDG predominate in the chloroplast envelope and the thylakoid membrane. Phosphatidylglycerol (PG) is specific to the thylakoid membrane. The nature of lipid classes as well as the acyl chain length varied greatly among different plant organs. For instance, Arabidopsis leaves and flowers have the highest levels of phospholipids while roots have the least [26]. PC, PE and PS are the phospholipids that contain significant amounts of VLCFAs. It has been demonstrated that these three phospholipids are composed mostly of 18-carbon species combined with a very long acyl chain ranging from 20 to 26 carbons.
PS is the lipid class that contains the highest proportion of VLCFAs. It can represent 69% of total PS found in roots whereas VLCFA-PC average only 1%. The amount of VLCFA-PE is also abundant in roots, while VLCFA-PS presents a slight increase in roots and a significant increase of PS in seeds. In the report of Millar et al., the authors demonstrated that the overexpression of seed specific KCS FAE1 increases the levels of VLCFAs in leaf glycerolipids where VLCFA-PC and PE can reach respectively 18% and 27% of phospholipids compared to 2 and 5% in WT [28].
Sphingolipids are also important structural components of membrane, accounting for more than 40% of plasma membrane lipids in some plants, but also found in the Golgi network and in endosomes [29]. They are composed of a long chain base (amino alcohol) and an amide linked fatty acyl chain. More complex sphingolipids are formed with the addition of a glycosylated polar head. Plant sphingolipids include four main lipid classes: the simple sphingolipid-ceramide and hydroxy-ceramide and the more complex forms, glucosylceramide and glucosylinositolphosphorylceramide. Plant sphingolipid diversity reflects their distribution among plant tissues. For instance, seed glucosylceramides are enriched with dihydroxy long chain bases and C16 to C20 saturated hydroxy-fatty acids while leaves are enriched with trihydroxy long chain bases and saturated very long acyl chains (C20 to C26) [30]. Sphingolipids show also structural specificity among organisms. Sphingomyelin, which has phosphocholine as the polar head group and is the major phosphosphingolipid in animals, has not been detected in plants. On the other hand, yeast contains exclusively inositolphosphorylceramides. Our knowledge of sphingolipid and glycerolipid functions are mainly learned from studies in mammals and yeast where both classes of lipids serve as structural membrane components and precursors of signaling molecules. Elevated levels of complex sphingolipids were associated with cell apoptosis, terminal differentiation, or cell cycle arrest [31]. The reduction of complex sphingolipid levels by contrast can lead to an increase of sphingoid long chain base leading to cell proliferation [32].
One of the first uncovered roles of sphingolipids in general concerned programmed cell death (PCD) as a mechanism of defence against pathogens. ASC-1 gene has been demonstrated to be homologous to LAG1 (longevity assurance life) and LAC1, which both encode the yeast ceramide synthase. Alternaria alternat f.sp. lycopersici is a host-selective toxin-producing fungus able to induce programmed cell death in asc/asc tomato plants. This process has been shown to correlate with a strong reduction of sphingolipids and increased levels of LCB (dihydrosphingosine [DHS] and 3-ketodihydrosphingosine [3-KDHS]), indicating that programmed cell death might be linked to sphingolipid synthesis [33].
Recently, Raffaele et al. showed the involvement of VLCFAs in the activation of the hypersensitive cell death response in Arabidopsis when they explored the role of MYB30 transcription factor during this process [34]. Two hypotheses were emphasized to explain the role of VLCFAs in the regulation of cell death: since the overexpression of MYB30 slightly modifies the wax composition, VLCFAs could compromise plant response to pathogens by modifying the cuticle [34]. Several studies suggested that cuticular waxes could directly inhibit pathogen growth or just provide a physical barrier to the pathogen entrance. However, as the overexpression of MYB30 results also in a larger change in VCLFAs containing sphingolipids, another hypothesis would be would be that VLCFAs sphingolipids could be involved in PCD in response to pathogen infection. VLCFAs sphingolipids could control PCD by structuring the plasma membrane allowing recognition of the pathogen and the initiation of the PCD signaling pathway. Alternatively, VLCFAs sphingolipids could act directly as signalling molecules as observed in animal apoptosis. Interestingly, the work of Reina-Pinto et al. showed that a misexpression of FAE1 in epidermal cells under the control of FDH promoter leads to the death of a specific cell type, which are the trichomes. However, this FAE1-dependent cell death was found to be sphingolipid-independent. An increase of long chain acyl-CoAs esters was observed in FDH:FAE1 plants and a chemical genetic screen indicated a role for phospholipase A2 and phospholipids, analogous to so-called “lipoapoptosis” observed in vertebrates [35]. Recently, the role of VLCFAs in plant cell proliferation has been highlighted by the further characterisation of the elongase dehydratase encoded by PASTICCINO2 gene (Fig. 2A). Indeed, pas2 exhibits severe developmental defects correlated with ectopic cell division, cell dedifferentiation and defective hormonal response, reminding one of the cell cycle defective phenotype observed in phs1 yeast mutant. Conversely, the overexpression of PAS2 in plants led to leaf serration and abnormal leaf development reminiscent of cell cycle inhibition and premature differentiation [1,36].
The role of sphingolipids in the deregulation of cell cycle and differentiation was also observed in animals and yeast. The lag1 gene was discovered in a screen for yeast longevity genes [37]. Furthermore, the Lass gene family, which are homologous to lag1 in animals, has been involved in breast cancer [38]. In vitro studies have correlated breast cancer progression with increased ceramide levels due to an upregulation of specific LASS genes. In particular, increased levels of 16:0-Cer, 24:1-Cer and 24:0-Cer were found in malignant tumors compared with benign tumors and normal tissue. Similarly, a study demonstrated that exogenous application of short chain ceramide led to the dephosphorylation of Retinoblastoma (Rb) gene, an event that blocks the cell cycle [39]. However, ELOVL4 deficient mice exhibited depletion in the epidermis of ceramides with very long chain fatty acids (C28+) and accumulation of ceramides with C26 fatty acids. This accumulation in VLCFAs-ceramides is correlated with defective skin permeability, leading to early postnatal death [40]. Altogether, these data indicate that LCFA/VLCFA-ceramides act as a rheostat controlling cell division and differentiation [41,42].
VLCFAs are also involved in cellular homeostasis via their involvement structural membrane lipids influencing membrane dynamics (membrane bending and fusion, membrane microdomains). For instance, C26 fatty acid, which is the major VLCFAs in yeast, has been demonstrated to be critical for membrane bending [43]. The isolation of a conditional acyl-CoA carboxylase mutant showed that reduced amounts of 26:0 lipids are linked to defects of the nuclear pore, mostly consisting in the separation of the inner and the outer membranes of the envelop. More precisely, these defects were the result of the absence of C26-phosphatidylinositol, which biophysical properties were associated with the stabilization of highly curved membrane domains [44]. The importance of the structural role of VLCFAs at the plasma membrane is also illustrated during cytokinesis. Cytokinesis in animals is carried out by the constriction of an actimyosin ring coupled to plasma membrane ingression. Szafer-Glusman et al. reported that the loss of function of the BOND gene, a member of the VLCFAs condensing enzyme Elovl blocks or strongly delays the cleavage furrow during early telophase in dividing Drosophila spermatocytes [45]. Furthermore, they showed that the bond phenotype differs from those of membrane trafficking mutants such as arf6 or rab11. Thus, the authors concluded that the BOND function might contribute to the cytokinesis by controlling the ability of membrane formation or bending during furrow ingression rather than being involved in membrane trafficking. In plants, a similar observation was made with FAE1 overexpressing plants that display an accumulation of VLCFAs in membrane glycerolipids associated with severe defects in plant morphology [27]. In particular, the authors noticed a highly curved thylakoid membrane. Millar et al. speculated that the accumulation of high levels of VLCFAs in the major plasma membrane lipids (PC and PE) might be responsible for the alteration of membrane curvature [27].
Sphingolipids are also contributing to the formation of membrane microdomains or lipid rafts. In mammals and yeast, these lipids rafts are involved in endocytosis, and protein sorting. In yeast, C26-ceramide-bound is critical for the routing of newly synthesized protein to the plasma membrane [46,47]. However, it has been recently demonstrated that yeast are still viable in the complete absence of sphingolipids [48]. In plants, reduction of CER10 activity led to strong VLCFAs reduction in all lipid classes (waxes, triacylglycerols, sphingolipids) causing cell expansion defects in epidermal cells and trichomes. The lack of cell expansion was correlated with reduced endocytosis. To discriminate between the different VLCFAs lipid classes, RNAi lines targeting CER10 in epidermal cells or in the embryo, led to specific reduction of 40% of wax load and 30% of seed triacylglycerols without any consequence on plant morphogenesis suggesting that sphingolipids were most probably involved in endocytic membrane dynamic associated with aberrant cell expansion [17].
6 Conclusion
Considerable progress in our understanding of VLCFAs has been made in the past 5–10 years mainly thanks to the isolation of the elongase mutants. Nevertheless, further investigations of the biophysical properties of VLCFAs will be needed to better understand their roles in cell physiology. It is clear now that, by being involved in such diverse lipid pools, VLCFAs are associated with very different cellular functions, cell proliferation, differentiation or death. The identification of the molecules involved in epidermal cell proliferation during postgenital organ fusion or cell death will be some of the challenges of the years to come. Convergent evolution is remarkable in a way that complete absence of VLCFAs in wax esters led to abnormal epidermal cell response, both in plants with cell proliferation during postgenital fusions and in vertebrates with cell death during skin development. In animals, increased levels of VLCFAs lead to several neurodegenerative diseases. For instance, studies demonstrated that autism was correlated with increased levels of plasma VLCFAs potentially due to impaired mitochondrial fatty acid oxidation [41]. X-adrenoleukodystrophy (ALD), which is a severe neurodegenerative disorder, is characterized by progressive demyelination within the central and peripheral nervous system. This disease is caused by a deficiency in Abcd1 peroxisomal transporter resulting in an impairment of peroxisomal beta-oxidation and the accumulation of saturated very long chain fatty acids in plasma, fibroblasts and tissues [42].
In plants, even if most of the elongase mutants present similar developmental defects (postgenital fusions, glaucous appearance, trichome defects), specific phenotypes are also observed such as cell proliferation for pas2 mutant, cell expansion defects for cer10 and absence of lateral roots for silenced-KCR1 plants. Although, these pleiotropic phenotypes could be due to different allele strength, we cannot exclude crosstalk with other pathways. Indeed, the presence of a protein tyrosine phophatase (PTP) motif in PAS2 protein led originally to the definition of PAS2 being a PTP-like protein involved in cell cycle regulation by interacting with cyclin dependent kinase A [49]. The conservation of this motif across eukaryotes suggests that phosphorylation dependent protein interaction and regulation might be involved in fatty acid elongation. The fact that mutation in PAS2 mammalian homolog PTPLA led to centronuclear myopathy that is reminiscent to phophosinositide phosphatase myotubularin MTM1 phenotype supports this hypothesis [1].
Functional genetics has opened the way to a better characterization of the enzymes involved in VLCFAs synthesis but many exciting challenges remain to understand the role of each lipid molecules, its cellular compartmentalization and its dynamics during growth and development.
Acknowledgements
We thank Brice Bourdenx for helpful discussions. We are grateful to Anne Marie Jaunet and Nelly Wolff (INRA Versailles) for their help in scanning electron microscopy. We acknowledge funding from the region Île-de-France (Bourses Cancérôple) and the ANR SphingopolaR (ANR-07-BLANC-0202).