1 Introduction
Xenopus oocyte was first demonstrated to have the capacity to translate exogenous mRNAs, then it was shown that this cell is particularly suitable for other studies such as electrophysiology, Ca2+ signalling, the developmental and cellular mechanisms of RNA localization, axis specification and establishment of the cytoskeleton changes during oogenesis. The latter aspect constitutes the topic of this review. Indeed, the stage-dependent cytoskeletal organization of the Xenopus oocyte is unique with respect to the oocyte of other Amphibian genders (Discoglossus, Bufo, Rana etc.); this peculiarity constitutes a rewarding model for the study of the cytoskeleton in relation to functional stages of oogenesis. In the Xenopus oocyte, the cytoskeleton distribution is dynamically regulated during oogenesis. During diplotene, oocytes undergo important functional and molecular changes related to oogenesis itself or to the development of the prospective embryo. Indeed, the occurrence of spatial distribution of organelles and molecules, which is of fundamental importance for the organization of the oocyte, strictly depends upon the cytoskeleton and viscoelastic conditions of the cytoplasm. An actin-based cytoskeleton is present in the cortex starting from early stage 1 [1–3], when the oocyte does not display clear signs of polarization in the cytoplasm (Fig. 1a, b). At the end of oogenesis, stages 4–6, a complex and polarized cytoskeleton network is present in the cytoplasm. As a result, a radial distribution of filaments extends from the cortex to the Germinal Vesicle (GV) periphery (Fig. 1a, b). In addition to actin, several cytoskeletal proteins have been detected in the growing oocyte, including tubulin [4–12], vinculin [13], vimentin [14–20], talin [1], cytocheratin [21–26], spectrin [26–29] and myosin [2,30–32]. When the oocyte reaches final stages of growth (stages 4–6)1, it acquires a defined polarization with respect to its virtual animal/vegetal axis (A/V axis). The eccentrically located germinal vesicle marks the animal side, and several cytoplasmic constituents, such as yolk platelets and ribosomes, distribute along the A/V axis (Fig. 1a, b). Immunocytochemistry coupled with disruptive drugs showed that actin, intermediate filaments, and microtubules have a fundamental role in the establishment and maintenance of this animal/vegetal polarity. In particular, in fully grown oocytes, cortical and perinuclear actin is linked to microtubules through their minus end, displaying the mechanical and functional scaffolding of the oocyte [33,34].
2 The early oocyte
2.1 Cytoskeleton organization
In stage-I oocytes, in which the yolk has not yet been deposited, the CSK forms a supple network that gradually spreads throughout the cell and is primarily composed of actin microfilaments, microtubules, vimentin, cytokeratin and spectrin [3,21,34,36]. Actin-binding spectrins of 239 and 100 kDa associate with the cell membrane, the nuclear envelope and the growing mitochondrial cloud (MC) [26,29], similarly to the organization achieved by the intermediate filaments (Fig. 1 and Fig. 2) [34,37]. Antibodies to β-spectrin identify a further isoform of about 230 kDa surrounding the nucleoli in the oocyte germinal vesicle (GV) [29]. It is known that protein 4.1 (4.1R), an 80-kDa polypeptide, stabilizes the spectrin–actin network and anchors it to the plasma membrane [38,39]. Immunoprecipitation experiments with antibodies directed against this protein indicated that at least two isoforms of the 4.1R protein are present in X. laevis oocytes: a 56-kDa form in the cytoplasm and a 37-kDa form in the GV, both isoforms associate with the 100-kDa isoform of β-spectrin. Moreover, the 56-kDa form coimmunoprecipitates with a cytokeratin of the same Mr. Double immunolocalization indicate that p4.1R colocalizes with actin in the cortex of oocytes as fluorescent dots [36], and forms with cytokeratin two separate networks that overlap throughout the whole cytoplasm (Fig. 3a, b). It was hypothesized that protein 4.1R could function as a linker protein between cytokeratin and the actin-based cytoskeleton [36].
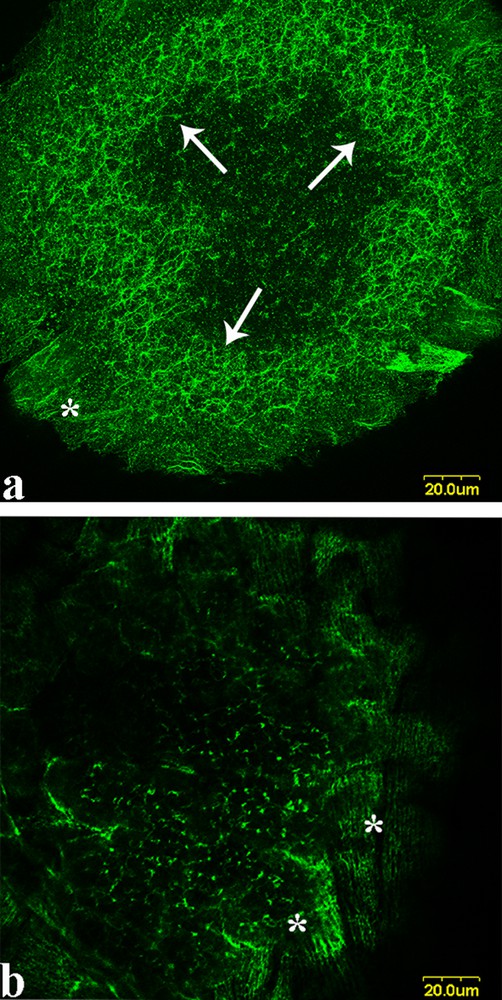
Cytokeratin distribution in early stage II oocyte of X. laevis; a: the CK forms a network in the oocyte cytoplasm (arrows); in follicular cells that surround the oocyte, the CK is distributed in form of subtle, parallel filaments (a and b, asterisks). For details see [36].

Confocal colocalization of p4.1R with cytokeratin in polarized X. laevis oocytes. p4.1R and CK were stained with polyclonal anti-p4.1R8/10 and monoclonal anti-CK antibodies, respectively. The secondary antibodies were BODIPY-conjugated anti-rabbit IgG (green) and Texas Red-conjugated anti-mouse IgG (red). Projection of ten sections perpendicular to the A/V axis of a polarized oocyte; a: animal half. Large areas of colocalization in the form of yellow dots are detected along CK filaments crossing p4.1R 8/10 filaments (arrows); b: vegetal half. The networks of p4.1R 8/10 and CK intersect and colocalize in large areas composed of spots (arrow). For details, see [36].
Therefore, in this early oocyte stage, the CSK is well present and in the process of gaining a more complex organization (Fig. 1b).
2.2 Cytoskeleton and RNA
It has been postulated that the CSK ensures that a critical mass of translation factors and RNAs comes in contact with each other to facilitate efficient translation [40–43].
In the early-stage oocyte, RNA transcription and translation are active, ensuring the synthesis of proteins essential for the oocyte itself and for the future embryo. In particular, proteins involved in steps of RNA maturation and in translation processes were found associated with spectrins, keratins, and tubulin [44]. Ribosomal subunits form in the nucleolus and are then exported to the cytoplasm, where they associate with a vast number of essential factors, a process particularly relevant in the X. laevis oocyte, which contains more than 1000 nucleoli [45] and associate with cytoskeletal proteins.
Information is available on proteins involved in 60S ribosomal maturation and/or translation in Xenopus stage I, such as rpl10, the eukaryotic initiation factor 6 (Eif6), thesaurins A/B and P0. rpl10 is necessary for the formation of an active ribosome, as it participates in the maturation of 60S [44]. Thesaurins are homolog of the eEF1-elongation factor in X. laevis oocytes [46]. P0 is the ribosomal stalk protein that binds to the GTPase centre and eEF1 during translation [47] by organizing the architecture of the aminoacyl-tRNA binding site on the ribosome [48]. Eif6 binds to the 60S ribosomal subunits in the nucleolus [49]. The transport of 60S from the nucleus to the cytoplasm has already been described in animal cells including Xenopus oocytes [50]. In preparation for 60S nuclear export, several proteins including Eif6 associate with pre-60S [47,51,52]. A large pool of Eif6 then localizes into the cytoplasm. Eif6 may bind to mature 60S, thus regulating 60S availability [53]; if protein kinase C phosphorylates Eif6 at serine 235, the affinity of Eif6 for 60S is reduced, and the availability of 60S for translation and the formation of the active 80S ribosome is increased [54]. Both eif6 and rpl10 associate with spectrin (Fig. 4), cytokeratin and tubulin, while thesaurins interact only with spectrin and cytokeratin [44]. Given that, upon ribosomal maturation, rpl10 inserts into the 60S subunit simultaneously with the release of eif6 [48]; it was hypothesized that actin-based CSK and microtubules provide the necessary scaffold for the insertion/release of these two molecules and, subsequently, for eif6 transport and binding to the mature 60S subunit. Thesaurins aggregate at the oocyte periphery, making this a favourable site for protein synthesis. In fact, the CSK may support the interaction between thesaurins and sites of the translating ribosome. Moreover, as the assembly of the ribosome stalk (where P0 is located) to the 60S subunit is essential for the release of eif6, it can be assumed that the CSK facilitates the binding of the stalk to the 60S [44]. These data support the hypothesis that cytoskeletal proteins have a specific functional role during ribosome maturation and translation.
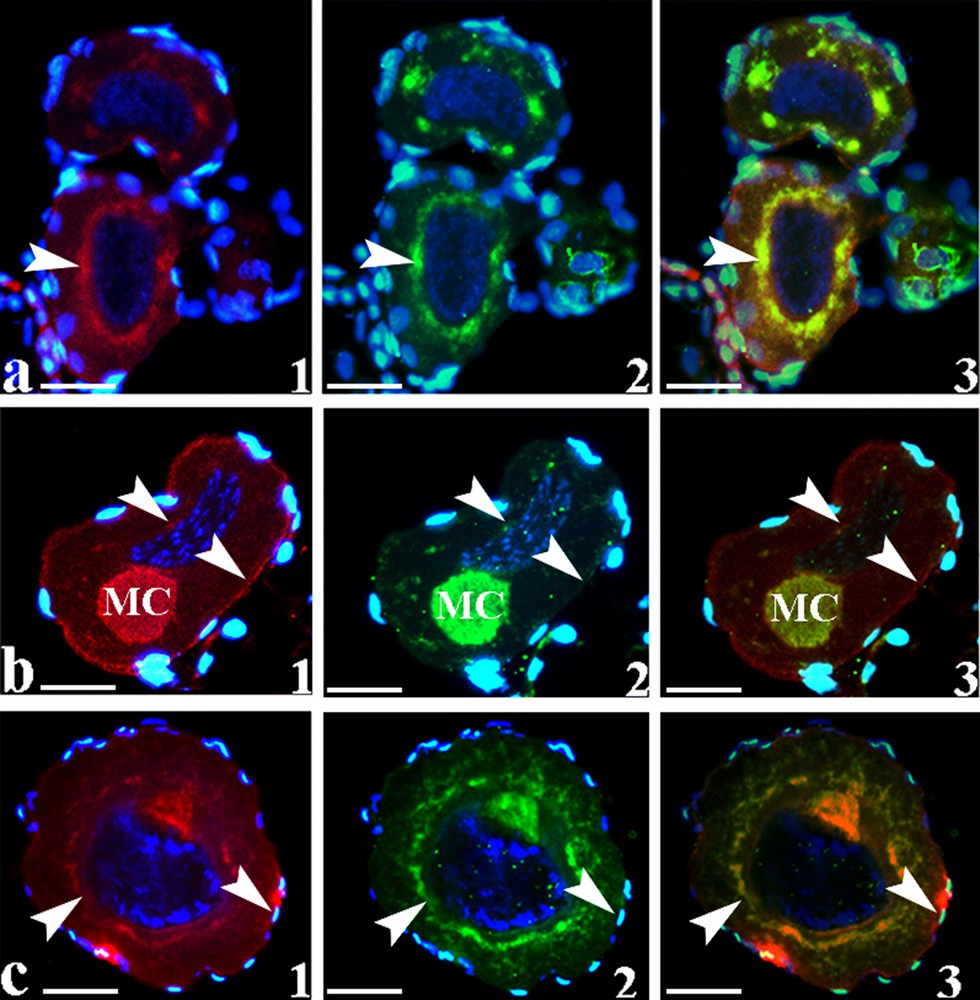
Immunofluorescence in stage I oocytes; a1, b1, c1: immunostaining with monoclonal anti-rpl10 revealed using anti-mouse IgG conjugated with Texas Red. The heavy fluorescent rim around the oocyte labels the somatic tissue that typically surrounds oocytes. The immunofluorescence was localized in the mitochondrial cloud (MC), around the nuclear envelope (arrowheads) and mildly at the oocyte periphery (arrowheads), similar to the immunolocalization of spectrin using polyclonal anti-spectrin; a2, b2, c2: and revealed using anti-rabbit IgG conjugated with BODIPY, in green; a3, b3, c3: colocalization of the two proteins in the MC and the nuclear envelope (arrowheads). Some immunostaining was also detected at the oocyte periphery (arrowheads) and in the cytoplasm. The sections were counterstained with DAPI. Bars = 21 μm.
3 Towards oocyte polarization: the mitochondrial cloud
At st.1, the definitive mitochondrial cloud (MC), rich in active mitochondria [55], grows anchored to the nuclear envelope, making up a clear sign of asymmetry in the oocyte. In fact, the virtual animal/vegetal axis of the oocyte can be traced because of the position of the MC (Fig. 5c) [56]. The MC can be considered as a package of RNAs held together by a network of cytoskeletal proteins, endoplasmic reticulum (ER) and mitochondria, used to transport genetic information to the oocyte cortex. Indeed, it contains germ plasm mRNAs (Xcat2, Xdaz1, Xpat, DEADSouth, etc.) in a region facing the plasma membrane called METRO (Fig. 5b) [57] and other molecules such as Xlsirts, Xwnt-11, Xotx1 mRNAs [58,59], cytokeratin [5,26,60,61], spectrin [26,57], γ-tubulin [12] and p4.1 [36]. The conspicuous network of cytoskeleton components surrounds permeates and sustains the MC [36,60,61]. The MC gradually migrates towards the vegetal cortex through an unknown mechanism. Indeed, the CSK cannot exert contractile activity because this ability is acquired later in oogenesis, after oocyte maturation [62]. Moreover, MC migration does not appear to be governed by a viscoelastic change in the region where it moves [63]. At stage II of oocyte growth, the MC releases its content into the proximal cortex, thereby marking it as the vegetal pole [58]. The mRNAs remain tightly anchored to the vegetal cortex, thanks to cytoskeletal proteins, until the end of oogenesis [64] (Fig. 1b and Fig. 5a, b).

mRNA localization in the early oocyte of X. laevis; a: oocyte stages I–II with evident MC (arrowhead), arrow indicate the nucleus; b: X-cat2 localization in the METRO area of MC (arrowhead); c: schematic view of the localization of XNOA 36 and α-spectrin mRNAs with respect to the virtual A/V axis, as determined by the position of the MC; d: in situ hybridisation on a sectioned specimen, showing XNOA 36 and α-spectrin mRNA localisation in stage-1 oocytes of about 270 μm. mRNA bisect the oocyte cytoplasm in two halves. XNOA 36 (1) as well as α-spectrin (3) mRNAs are labelled by BM purple staining. Both mRNAs are segregated in the half of the oocyte opposite to the hylum (asterisk). The MC (arrowhead) is positioned at the boundary between the labelled and unlabelled regions of the oocyte. The bar in 1 indicates 58 μm, and 63 μm in 3. (2) double label in situ hybridisation showing the localisation of XNOA 36 mRNA (detected through BM-purple staining) and α-spectrin mRNA (detected through Vector black). The two hybridization signals overlap (reddish-purple staining) and sites of co-localisation are seen, in particular around the MC (arrowhead). Bar = 52 μm. (4) Detail of panel 2 showing, at higher magnification, the hybridisation signals at the MC periphery (arrow). Bar = 10 μm. For details, see [26].
In Xenopus, α-and β-spectrins (mRNA and proteins) are segregated in a cap located at the oocyte cortex. This cap is detectable during the translocation of the MC to the cortex and constitutes an early event of cytoskeleton polarization [65]. Indeed, α-spectrin mRNA marks this transient asymmetry already in stage-I oocytes and co-localizes with the mRNA of XNOA 36, homologous to mammalian NOA 36 RNA. The latter is present in the nucleolus in G1/G2 or associated with centromeres during metaphase in mammalian cells [66]. In X. laevis oogenesis, both XNOA 36 and α-spectrin mRNAs undergo a gradual segregation in the oocyte, parallel to the A/V axis, thereby virtually cutting the MC and the oocyte in two halves (Fig. 5c, d1–4). Moreover, XNOA 36 mRNA co-localises with the α-spectrin protein in thin filamentous structures and co-immunoprecipitates with this protein. Therefore, the spectrin-containing cytoskeleton, i.e. the actin-based cytoskeleton [67–69] may account for the network decorated by XNOA 36 mRNA. These findings suggest the existence of a cytoskeletal-dependent process of RNA localisation/anchoring in stage-I oocytes of Xenopus laevis. In addition, the cytoskeletal network where XNOA 36 mRNA is located appears to hold the MC during its migration to the cortex. It was hypothesized that the asymmetric withdrawal of the cytoskeleton containing XNOA 36 mRNA might supply the force needed to drag the MC towards the cortex [70]. Importantly, the described localization pattern of the spectrin mRNA and protein is transient and it is no longer detected in that pattern in oocytes after growth stage I. Moreover, the MC region METRO rich in mitochondria appears to contain the XNOA 36 protein (Fig. 5d4) that is also noticeably present in the vegetal cortex in front of the MC [70]. The prediction of XNOA 36 subcellular localization, confirms that it is at least 4.3% cytoskeletal and 4.3% mitochondrial [26]. As the cortex is notoriously rich in cytoskeletal proteins [64] including spectrin [26], it was hypothesized that the centromeric XNOA 36 protein interacts with the cytoskeleton, participating in the anchorage of the MC and its mRNAs in the future vegetal pole [70].
4 Stages II–III
In these stages, pigment granules accumulate and yolk synthesis proceeds from the oocyte periphery. Following the release of the MC content into the vegetal cortex, at stages II–III, a second pathway (“the late pathway”) transports to the region marked by the MC content mRNAs such as Vg1 and VegT mRNAs, responsible for endoderm and mesoderm specification [45]. Notoriously, the oocyte does not have centrosomes. Interesting studies sustain that [57] the MC contains and releases residual centriole proteins such as γ-tubulin, which could be used in the late mRNAs pathway as a microtubule-organizing centre (MTOC). Indeed, the MC content appears to be the centre of organization for a microtubule array that is involved in the transport of Vg1 and VegT mRNAs to the future vegetal half of the oocyte [56,71–73]. This pathway occurs when the Vg1 and VegT mRNAs are found in a bell-shaped structure rich in ER vesicles located in the stream of MC migration. The mRNAs probably associate with the vesicles that migrate along the MT orientated toward the vegetal pole. Thus, the mRNAs arrive in the territory marked by the MC content [74]. Gradually, the MTs reorganize in the whole vegetal hemisphere of the oocyte and later associating CSK organization spread in the whole cytoplasm. It was also hypothesized that, as in mammals XNOA 36 associates with centromeres [66], in Xenopus oocyte this protein may associate with the residual centriole proteins of the MC participating in early microtubules reorganization. The process starting at the MC disaggregation site in the vegetal cortex, spreads to the rest of the oocyte [61] cortex, where XNOA 36 is also located (Fig. 5d) [26]. In this transitional phase of oogenesis, α-spectrin RNA and other CSK proteins gradually gain a new organization in the oocyte. In particular, confocal immunolocalization shows that in the cytoplasm of the oocytes, a loose network of fibres stained by anti-4.1R antibodies spreads across the cytoplasm (Fig. 3a, b [36]. The CSK is abundantly present also in the follicle cells, where it forms a conspicuous cytoskeletal network (Fig. 2a, b; asterisks).
5 Stages IV–VI
Stages III–IV are intermediate stages of reorganization of mRNAs and proteins, and, only during stages IV–VI, the oocyte acquires its final symmetry displaying a radial organization along the A/V axis. Microfilaments and microtubules radiate from the nuclear envelope to the oocyte periphery (Fig. 1b) [60].
Stage IV–VI Xenopus oocytes contain an extensive network of cytoplasmic microtubules [12] and a substantial pool of the centrosomal protein γ-tubulin surround the GV, indicating that the GV serves as a MTOC in late oogenesis. γ-Tubulin is also concentrated in the cortex, its distribution being polarized along the animal-vegetal axis. γ-Tubulin is associated with MTs in the cortical cytoplasm. The distribution of this protein was not significantly affected by either cold or nocodazole, but was partially disrupted by cytochalasin B [12], suggesting that γ-tubulin may serve as a link between the oocyte MT network and cortical actin. Therefore, the distribution of cortical γ-tubulin temporally coincides with the formation of the A–V axis and the polarization of the oocyte MT cytoskeleton during stage IV/V of oogenesis. A similar polarization of the CK filament network occurs in mid stage IV-stage V, and eventually the filament networks adopt the above-mentioned characteristic organization of stage VI oocytes (Fig. 1) [34].
The cytoplasmic localization of α-spectrin indicates a different organization of the cytoskeletal network in the animal and in the vegetal halves, resembling the mentioned distribution of tubulin and cytokeratin in the oocytes at the same stages. In fact, immunofluorescence reveals that in the cytoplasm, α-spectrin is organized in the form of radii in the animal hemisphere, while in the vegetal half, it is apparently less orderly organized [65]. Moreover, in the oocyte periphery, spectrin mRNA shows a specific regionalized distribution, as it accumulates at the periphery of the oocyte animal half. Importantly, spectrin has binding sites for actin, tubulin, intermediate filament and p4.1R [36,75,76], and its presence in the cortical cytoskeleton may determine domains related to the distribution of specific plasma membrane proteins [68]. The segregation of α-spectrin mRNA in the animal half can be of relevance because in this region during oogenesis and maturation, the oocyte acquires the ability of fusing with sperm [65]. α-Spectrin may anchor plasma membrane molecules involved in sperm binding and fusion [77].
p4.1R immune-localization reveals a network, particularly in the peripheral cytoplasm, which is most evident in the vegetal half of the oocyte. The underlying network is looser, leaving open areas about 30 mm wide. Confocal microscopy reveals that this network is made of single filaments (thin filaments) and bundles of filaments (thick filaments). Thin filaments form a tighter network than thick filaments do. In addition, both filaments partially co-localize with CK in the form of spots that are differently associated according to which hemisphere they are located in (Fig. 3 a, b) [36]. The β-spectrin network is distributed in similar regions and is tighter than that formed by p4.1R. Although there is no direct evidence for an interaction between p4.1R and spectrin, co-immuno-precipitation experiments suggest the presence of co-localization sites. This presumptive interaction might contribute to the stabilization of structural domains both in the peripheral cytoplasm and next to the plasma membrane [36].
6 Concluding remarks
About twenty years ago the cytoskeletal organization of Xenopus laevis was described in detail [9–12,24,25,33,34,37,60,61]. The conclusion of those studies was that Xenopus oocytes are characterized by a complex filament cytoskeleton composed of microtubules, microfilaments made of actin and associated proteins and of intermediate filaments [34]. Intermediate filaments are the last of the three major cytoskeletal networks (F-actin, MTs, and CK filaments) established in Xenopus oogenesis. This observation and the use of CSK-disruptive drugs suggested that the organization of the oocyte CSK depends upon a hierarchy of interactions among F-actin, MTs, and CK filaments. The authors concluded that the cortical CK network appeared to be highly dependent upon F-actin microfilaments and MTs. MTs were anchored to cortical and perinuclear actin via γ-tubulin. CK filaments were anchored to actin in the cortical and perinuclear cytoplasm by an unidentified protein. Cytokeratins could be linked to MTs trough a MT-dependent motor protein [34]. More recently, Carotenuto et al. (2009), while studying p4.1R in Xenopus oogenesis, have showed that both the 37- and 56-kDa isoforms of protein 4.1R associate with the 100-kDa isoform of β-spectrin. Moreover, the 56-kDa isoform of p4.1R coimmunoprecipitates with a cytokeratin of the same molecular weight, leading to suppose that p4.1R may very well constitute a natural linker between the actin cytoskeleton and cytokeratins. Indeed, confocal immunolocalization of protein 4.1R and cytokeratin depicts two separate networks that overlap throughout the whole cytoplasm.
The role of CSK in sustaining and collocating the oocyte constituents have further important functional consequences. Indeed, it was postulated that CSK ensures that a critical mass of translation factors and RNAs comes in contact with each other to facilitate efficient translation [40–43]. In Xenopus, early stages of oogenesis are scenarios of fundamental processes of RNA maturation and protein synthesis. The oocyte cytoskeleton scaffold binds to organelles and molecules, such as ribosomal 60S, polysomes, mRNAs and other factors including translation initiation and elongation factors. According to Chierchia et al. [44], important actors of these processes, such as the eukaryotic initiation factor 6 (Eif6), ribosomal rpl10, thesaurins A/B, homologs of the eEF1-elongation factor, and P0, the ribosomal stalk protein, appear to bind specifically spectrin, cytokeratin or tubulin, strongly suggesting that cytoskeletal components may support proteins related to discrete steps of RNA maturation and translation processes.
The cytoskeleton is also strongly involved in the determination of the oocyte polarity. In studying the role of the centromeric protein XNOA 36, it was found that in Xenopus oogenesis α-spectrin/XNOA 36 mRNAs and the onset of the Animal/Vegetal axis of st. I oocyte have a potential role. At this stage, a CK network containing α-spectrin mRNA and protein as well as XNOA 36 mRNA transiently segregates in one half of the oocyte, with the MC located between the XNOA 36/α-spectrin mRNA-labelled and unlabelled regions. This localization parallel to the virtual A/V axis may represent a transient indication of symmetry in the oocyte. Therefore, these findings suggest the existence of a novel cytoskeletal-dependent process of RNA localisation/anchoring in stage I oocytes of Xenopus laevis. It was also speculated that the asymmetric withdrawal of the cytoskeleton containing XNOA 36 mRNA might supply the force needed to drag the MC towards the cortex in late stage I.
At stage II of oocyte growth, the MC releases its content into the proximal cortex, thereby marking it as the vegetal pole [58]. The MC mRNAs remain tightly anchored to the vegetal cortex, thanks to cytoskeletal proteins including spectrin [64,70]. Moreover, the MC appears to contain the XNOA 36 protein that is also abundantly present in the vegetal cortex in front of the MC [70]. It was hypothesized that centromeric XNOA 36 protein may interact with the cytoskeleton, participating in the anchorage of the MC mRNAs in the future vegetal pole [70].
At stage III, the arrangement of MT radiating from the vegetal cortex to the GV is an impressive example of transportation of mRNAs (Vg1 and VegT) mediated by tubulin. The mRNAs localize at the vegetal pole and the following fertilization will specify the mesoderm and the endoderm of the future embryo [45]. The radial organization of the CK radii in the fully-grown oocyte takes place starting from this site.
Role of the funding source
This work was supported by a grant from the University of Naples Federico II–Ricerca Dip. 2017.
Disclosure of interest
The authors declare that they have no competing interest.
Acknowledgements
The authors wish to thank Dr. Maria Carmela Vaccaro and Dr. Nadia De Marco for suggestions.
1 Staging of the Xenopus oocytes. The growth stages of the X. laevis oocyte are: stage I (50–300 mm), stage II (300–450 mm), stage III (450–600 mm), stage IV (600–1000 mm), stage V (1000–1200 mm), and stage VI (1200–1300 mm) according to [35].