1 Introduction
Production of ethanol and other low-priced products from lignocellulosic biomass is currently of great interest [1]. One of the major limitations of the second generation (cellulosic) ethanol production is the hydrolysis step [2], where fermentable sugars are released from biomass using enzymes. This process step has to be further improved before commercialisation of the process. There are several approaches to increase the efficiency and decrease the costs of cellulose hydrolysis, i.e. better pretreatment techniques [3,4], optimising cellulase enzyme complex composition [5], genetic modification of cellulolytic microorganisms [6], tailoring enzymes for better performance [7] or addition of proteins, surface active additives (also called surfactants) and other chemicals to enhance cellulose conversion.
Surfactants have already been recognised as advantageous additives in cellulase production [8,9] in the late sixties by increasing the cell wall permeability and releasing cell-wall bound enzymes to the liquid phase. It was found later [10] that surfactants prevent cellulases from surface inactivation. In the past three decades, numerous studies have investigated the adsorption of cellulases and the effect of surfactants and other additives on hydrolysis of cellulosic and lignocellulosic substrates (Table 1). Using various additives the cellulose conversion has significantly increased and the adsorption of cellulases has obviously decreased. It should be noted that the effect was more pronounced in case of substrates containing lignin than on substrates containing cellulose alone.
Results of enzyme adsorption experiments and reported effects of surfactant addition on enzymatic hydrolysis of various cellulosic and lignocellulosic substrates. The major hydrolysis parameters are also presented.
Substrate | S conc. [%] | Temp. [°C] | Time [h] | Enzyme | Additive type and concentration | Conversion [% of theoretical max.] | Enzyme adsorption | Ref. |
Avicel | 2 | 45 | 72 | 40 FPU/g cellulose Celluclast: Novozym 188 (1 mg: 0.25 mg) | - | ∼100 | Protein: ∼0% | [11] |
2 | 45 | 48 | 20 FPU/g cellulose Celluclast 1.5L: Novozym 188 (1 FPU: 1.75 CBU) | - | 82 | Protein: ∼15% | [12] | |
5 | 50 | 72 | 1 mg/mL Cellusoft L | Tween 20, 0.1% | 13→19 | n.r. | [13] | |
2 | 50 | 72 | 15 FPU/g cellulose Celluclast 1.5L: Novozym 188 (1 FPU: 1.75 CBU) | BSA, 1% | 75–75 | n.r. | [14] | |
5 | 40 | 96 | 1 mg/mL T. viride (Meicelase CEPB) | Tween 20, 0.05% | ∼35→∼44 | n.r | [15] | |
2.5 | 40 | 72 | Celluclast 1.5L: 0.69 FPU/mL Novozym 188: 1.40 IU/mL |
PEG 4000, 2.5 g/L | 67→75 | n.r. | [16] | |
2.5 | 50 | 72 | Celluclast 1.5L: 0.69 FPU/mL Novozym 188: 1.40 IU/mL |
PEG 4000, 2.5 g/L | 71→81 | n.r. | [16] | |
5 | 50 | 48 | Celluclast 1.5L: 15 FPU/g glucan Novozym 188: 30 CBU/g glucan |
PEG 4000, 0.05 g/g | 41→79 | FPA: 92%→3% BG: < 18% |
[17] | |
Sigmacel 100 | 2.5 | 50 | 96 | 40 IU/g T. viride cellulase (Sigma) | Tween 80, 4 g/L | 7→43 | n.r. | [18] |
Newspaper | 5 | 45 | 48 | 0.641 FPA/mL T. reesei QM 9414 enzyme (produced on Solka Floc) | Tween 80, 0.1% | 41→55 | FPA: 85%→67% | [19] |
0.5 | 50 | 48 | 10 FPU/g substrate, T. viride (IFO No. 30498) | POG(20) nonylphenyl ether 0.05 g/g substrate | 22→35 | FPA: 70%→60% | [20] | |
Acid pretreated corn stover | 2 | 50 | 72 | 15 FPU/g cellulose Celluclast 1.5L: Novozym 188 (1 FPU: 1.75 CBU) | BSA, 1% | 82→91 | FPA: 80%→50% BG: 95%→30% |
[14] |
Acid pretreated wheat straw | 5 | 50 | 24 | 5 FPU/g DM (Celluclast 1.5L + Novozym 188 5 mL: 1 mL) | BSA, 0.05 g/g DM | 16→74 | Endoglucanase: 94%→∼80%% | [21] |
AFEX corn stover | 2 | 50 | 72 | 15 FPU/g cellulose Celluclast 1.5L: Novozym 188 (1 FPU: 1.75 CBU) | BSA, 1% | 76→82 | n.r. | [14] |
Lime pretreated corn stover | 5 | 50 | 72 | 5 FPU/g biomass Spezyme-CP 28.4 IU/g biomass Novozym 188 |
Tween 20, 0.15 g/g biomass | 60→85 | n.r. | [22] |
Ethanol pretreated mixed softwood | 2 | 45 | 48 | 10 FPU/g cellulose Celluclast 1.5L + 20 IU/g cellulose Novozym 188 | - | 95 | Protein: ∼50% FPA: 60% |
[23] |
SP Douglas fir | 2 | 45 | 48 | 20 FPU/g cellulose Celluclast 1.5L: Novozym 188 (1 FPU: 1.75 CBU) | - | 43 | FPA: 59% BG: 62% |
[12] |
Delignified SP Douglas fir | 2 | 45 | 48 | 20 FPU/g cellulose Celluclast 1.5L: Novozym 188 (1 FPU: 1.75 CBU) | - | 99 | FPA: 17% BG: 27% |
[12] |
SP Douglas fir | 2 | 50 | 72 | 20 FPU/g cellulose Celluclast 1.5L: Novozym 188 (1 FPU: 1.75 CBU) | BSA, 1% | 54→73 | n.r. | [14] |
SP poplar | 1.25 | 50 | 96 | 40 IU/g T. viride cellulase (Sigma) | Sophrolipid, 1 g/L | 67% increase | n.r. | [18] |
SP lodgepole pine | 5 | 45 | 48 | 20 FPU Celluclast 1.5L 40 IU Novozym 188 |
Tween 80, 0.2% | 69→91 | n.r. | [24] |
SP spruce | 5 | 40 | 96 | Celluclast 2L: 0.66 FPU/mL Novozym 188: 0.81 IU/mL |
Tween 20, 0.25% | 56→73 | Cel7A: 73%→61% | [25] |
5 | 40 | 72 | Celluclast 1.5L: 0.69 FPU/mL Novozym 188: 1.40 IU/mL |
PEG 4000, 2.5 g/L | 57→82 | n.r. | [26] | |
5 | 50 | 72 | Celluclast 1.5L: 0.69 FPU/mL Novozym 188: 1.40 IU/mL |
PEG 4000, 2.5 g/L | 42→81 | n.r. | [26] | |
2 | 50 | 72 | Celluclast 1.5L: 20 FPU/g DM Novozym 188: 40 FPU/g DM |
PEG 4000, 2.5 g/L | 81→96 | Prot: 96% →60% FPA: 95%→ 44% BG: 57%→ 33% |
[27] |
Several surfactants have been screened for their ability to increase cellulase production [9,10] and enhance cellulose conversion [16,18,21,25,26]. Non-ionic surfactants were found to be the best ones [25]. When surfactants and polymers with various amount of ethylene oxide (EO) content were compared in enzymatic hydrolysis of steam pretreated spruce, increasing conversion was obtained with longer EO chains on the non-ionic surfactants [16]. Similar results were obtained by using only the hydrophilic part of the surfactant, the poly(ethylene glycol) (PEG) [26], which suggests that the hydrophobic part of surfactant is not solely responsible for the positive effect, but interactions between EO polymer and the substrate are dominating. Experiments with PEG, which is an amphipilic EO polymer with a molar mass less than 20 kDa, showed that addition of the chemical increases the conversion of steam pretreated spruce, especially at elevated temperatures [16,26] and has been shown to reduce enzyme adsorption on the surface of the lignin [16].
The role of lignin in the enzymatic cellulose breakdown has been verified by several authors. Sewalt et al. [28] have found a 14–60% decrease in cellulose conversion caused by addition of up to 15% lignin preparations to the hydrolysis. Boussaid and Saddler [11] have observed that cellulases were inactivated to a higher extent during hydrolysis of lignocelluloses (Douglas fir pulps) than during breakdown of microcrystalline cellulose substrate (Avicel). Lu et al. [12] have reported similar results using steam pretreated Douglas-fir substrate. Eriksson et al. [25] have proposed a mechanism about the role of lignin in the enzyme adsorption: the surfactants prevent non-specific binding of enzyme to the hydrophobic lignin on the surface of the lignocellulose substrate. Enzymes could bind unproductively to the lignin surface; therefore the amount of active enzymes and thus the hydrolysis rate are decreased.
Adsorption of cellulases during hydrolysis has already been tested on various substrates [11,12,23], and the decrease of adsorption in the presence of additives has also been demonstrated [14,16,21,25–27]. It was found that enzymes adsorb on the substrates to different extent. Enzymes can adsorb on lignocelluloses productively (on cellulose) and unproductively (on lignin). In the initial phase of the hydrolysis, when considerable amount of cellulose is present, both phenomena can be observed. Later, when cellulose is broken down into soluble sugars, the decrease of enzyme activity is rather dependent on the unproductive binding of the enzymes. Therefore more significant decrease of the enzyme activity can be observed in the first hours of hydrolysis [11,12,23,27,29]. Surfactants [19–21,25], BSA [14] and PEG [16,26,27] were found to be effective in the reduction of unproductive binding and different adsorption affinity of various enzymes were observed. According to Börjesson et al., the cellulose binding module has an important role in the unproductive binding of the enzymes [16].
As the adsorption behaviour of the enzymes on various substrates was different, obvious to explain these differences with the lignin structure. Sewalt et al. [28] have studied the inhibiting effect of different lignin preparations on cellulose degradation, and have found that unmodified lignins were more detrimental than hydroxypropylated lignins, and organosolv lignins than ones obtained with steam explosion. Berlin et al. [30] have also observed that the lignin isolation method had an impact on the inhibitory effect on cellulases, when different lignin preparations were isolated from the same source.
The impact of reducing the unproductive enzyme binding is not only the intensification of cellulose breakdown by increasing the conversion and decreasing the process time, but also giving the possibility for enzyme recycling by using the supernatant in an ongoing hydrolysis [23] or after ultrafiltration [31] which could further improve the process economy.
The aim of the present study was to test the enzyme adsorption in enzymatic hydrolysis of various steam preteated lignocelluloses (spruce, willow, hemp, corn stover, wheat straw, sweet sorghum bagasse) and its prevention by PEG addition. As significant differences were observed among the enzyme binding capacities on different substrates, the structure of the steam pretreated lignins has been analysed in order to find a connection between enzyme adsorption, its decrease by PEG addition, and chemical properties of steam pretreated lignins were investigated.
2 Materials and methods
2.1 Substrates
Six steam pretreated substrates were used in the hydrolysis experiments; one from softwood (spruce), one from hardwood (willow), and four perennial crop residues (wheat straw, hemp, sweet sorghum bagasse and corn stover). Steam pretreatments were performed at Department of Chemical Engineering, Lund University (Lund, Sweden) and ENEA, Italy (corn stover). Pretreatment conditions are summarised in Table 2. The substrates were rinsed with triple amount of warm (∼60 °C) distilled water to remove much of the soluble compounds.
Steam pretreatment conditions.
Raw material | Reactor type | Impregnation | Temp (°C) | Residence time (min) | Ref. |
SP spruce | Batch | 2.5% SO2 | 210 | 5 | [32] |
SP willow | Batch | 2% SO2 | 190 | 12 | [33] |
SP wheat straw | Batch | 0.2% H2SO4 | 190 | 10 | [34] |
SP hemp | Batch | 2% SO2 | 205 | 5 | [35] |
SP corn stover | Flow-through | - | 210 | 5 | - |
SP sweet sorghum bagasse | Batch | 2% SO2 | 190 | 10 | [36] |
2.2 Enzymes and chemicals
Enzymes were received from Novozymes A/S (Bagsværd, Denmark). Celluclast 1.5L had an overall cellulase activity of 88.8 FPU/mL, and β-glucosidase activity of 38.6 IU/mL. Novozym 188 had 408 IU/mL β-glucosidase activity.
Poly(ethylene glycol) (PEG) with an average molar mass of 4000 Da was obtained from Merck (Hochenbrun, Germany).
2.3 Enzyme activity assays
Filter Paper Activity (FPA) measurement was carried out according to Mandels et al. [37], with the modification, that an enzyme dilution releasing 1 mg glucose was used instead of 2 mg. To keep the pH at 4.8, sodium acetate buffer (0.05 M) was used.
β-glucosidase activity was measured according to Berghem and Petterson [38] with some modification. The substrate was 5 mM 4-nitrophenyl-β-d-glucopyranoside (pNPG). One millilitre pre-incubated substrate was mixed with 0.1 mL diluted enzyme solution and incubated for 10 min at 50 ̊C. The reaction was terminated by addition of 2 mL of 1 M Na2CO3 solution and then diluted with 10 mL distilled water. The amount of the liberated 4-nitrophenol was measured at 400 nm against blank.
Total protein content in the hydrolysis supernatant was measured according to Bradford [39] using Coomassie Brilliant Blue dye (Coomassie Blue G250, Sigma-Aldrich, St. Louis, MO, USA) with BSA as a standard.
2.4 Enzymatic hydrolysis
Hydrolysis experiments were performed in 100 mL screw-capped bottles using 2% dry matter (DM) substrate concentration and 50 mL reaction volume. Enzyme loading was 20 FPU/g DM substrate Celluclast 1.5L and 40 IU/g DM Novozym 188. The pH was maintained at 4.8 by sodium acetate buffer. Hydrolysis experiments were performed at 50 °C in water bath using magnetic stirrers at 250 rpm. Hydrolysis of SP spruce was also performed without β-glucosidase supplementation (abbreviated as SP spruce (Cel), while as SP spruce (Cel + Nov) when both Celluclast and Novozym were used). Hydrolysis was performed in triplicates with and without addition of 2.5 g/L PEG 4000.
2.5 Determination of protein adsorption and free residual enzyme activities
Total protein concentration and residual enzyme activities (FP and β-glucosidase) were measured in the hydrolysis supernatants in order to quantify the protein adsorption and the free residual enzyme activities. Prior to analysis, 15 mL of each sample was concentrated, using an ultrafiltration device (Vivaspin 4 centrifugal filter unit with 10 kDa PES membrane, Sartorius, Göttingen, Germany) and “washed” with buffer (5 mL, 0.05 M Na-acetate, pH 4.8) to remove sugars and other small molar mass compounds that interfere with enzyme activity measurements.
2.6 Compositional analysis
Lignin and carbohydrate contents of raw and pretreated materials were analyzed using NREL protocol [40] with some modification. The amount of oven dried (105 °C) sample was 0.5 g that was hydrolyzed with 2.5 mL 72% sulphuric acid at room temperature for 2 hours. After the reaction time was reached, this mixture was diluted with 72.5 mL distilled water and the hydrolysis was continued at 121 °C for 60 min. The samples were filtered and washed with distilled water through G4 glass filter crucibles. The remaining lignin on the filter was dried at 105 °C, weighed and placed in furnace at 550 °C for 6 hours in order to determine the ash content. The Klason lignin content was taken as the ash free residue after acid hydrolysis.
2.7 Pyrolysis gas chromatography/mass spectrometry (Py-GC/MS)
Approximately 0.6 g samples were pyrolysed at 600 °C for 20 s in helium atmosphere using a Pyroprobe 2000 pyrolyzer interfaced to an Agilent 6890A/5973 gas chromatograph/mass spectrometer. The pyrolysis products were separated on an HP-5MS capillary column (30 m × 0.25 mm, 0.25 μm film thicknesses). The GC oven was programmed to hold 40 °C for 2 min and then increase the temperature to 300 °C (hold 5 min) at a rate of 6 °C/min. The mass spectrometer was operated in the electron impact mode at 70 eV electron energy. The mass range m/z 14–500 was scanned by the mass spectrometer.
2.8 31P-NMR
Steam pretreated samples were prepared as previously described [41]. Accurately weighed samples of lignin (30 mg) were dissolved in a solvent mixture composed of pyridine and deuterated chloroform, 1.6:1 v/v ratio (0.4 mL). The derivatisation agent, 2-chloro-4,4,5,5-tetramethyl-1,3,2-dioxaphospholane (Aldrich, 0.1 mL), the internal standard (N-hydroxynaphthalimide) and the relaxation reagent (chromium(III) acetylacetonate) solution (0.1 mL), were then added. Quantitative 31P NMR spectra were obtained on a Bruker 300 NMR spectrometer by using previously described methods [42,43]. To improve resolution, a total of 256 scans were acquired. The 31P NMR data reported are averages of three phosphitylation experiments followed by quantitative 31P NMR acquisition. The maximum standard deviation of the reported data was 2 × 10−2 mmol/g, while the maximum standard error was 1 × 10−2 mmol/g.
2.9 HPLC
Liquid samples from raw material analysis and enzymatic hydrolysis upon their monomer sugar concentrations were determined with a Shimadzu HPLC system (Shimadzu, Kyoto, Japan) using an Aminex HPX-87H column (Bio-Rad, Hercules, CA, USA) at 65 °C. The eluent, 5 mM H2SO4 was used at a flow rate of 0.5 mL/min. Concentration of carbohydrates was detected upon their refractive index. All samples were filtered through a 0.22 μm pore size filter before analysis to remove solid particles.
2.10 Statistical analysis
Statistical evaluation of the results obtained was performed using Statistica 9.0 software (Statsoft Inc., Tulsa, OK, USA).
3 Results
3.1 Composition of various steam pretreated substrates
The compositions of the different steam pretreated lignocellulose substrates showed large variations, especially regarding their residual hemicellulose and lignin content (Table 3). Substrates from perennial plants had lower lignin content, with wheat straw as an exception, while woody materials and wheat straw had remarkably higher lignin content. The amount of hemicellulose present in the substrate may give information about the severity of the pretreatment, as the more severe the pretreatment is, the better the hemicellulose solubilisation in acid catalysed steam pretreatment.
Composition of steam pretreated substrates and cellulose conversions obtained in enzymatic hydrolysis. Mean values of triplicate measurements and standard deviations are presented.
Cellulose [%] | Hemicellulose [%] | Lignin [%] | Conversion [%] | Conversion with PEG [%] | |
SP spruce (Cel) (Cel + Nov) | 62.2 ± 0.2 | 3.2 ± 0.1 | 33.6 ± 1.1 | 59.5 ± 0.0 | 76.6 ± 1.1 |
78.8 ± 2.3 | 83.5 ± 0.4 | ||||
SP willow | 60.1 ± 2.5 | 4.4 ± 0.2 | 30.5 ± 0.3 | 96.9 ± 1.4 | 99.7 ± 1.9 |
SP wheat straw | 55.7 ± 0.9 | 2.5 ± 0.1 | 44.4 ± 0.2 | 99.5 ± 2.7 | 99.3 ± 0.7 |
SP hemp | 65.2 ± 0.8 | 8.0 ± 0.1 | 24.7 ± 0.2 | 83.1 ± 1.5 | 86.2 ± 0.7 |
SP corn stover | 48.2 ± 0.6 | 15.2 ± 0.2 | 20.3 ± 0.3 | 77.6 ± 2.0 | 76.0 ± 2.5 |
SP sweet sorghum bagasse | 58.3 ± 0.7 | 13.7 ± 0.4 | 19.5 ± 0.3 | 73.9 ± 1.5 | 76.4 ± 1.6 |
3.2 Effect of PEG addition on cellulose conversion
Besides the evaluation of the PEG effect on the cellulose conversion of various steam pretreated lignocellulosic substrates, the main focus of these experiments was the investigation of enzyme adsorption, therefore low substrate concentration and relatively high enzyme loading was applied. The 72-hour cellulose conversions achieved in hydrolysis experiments are shown on Table 3. A significant increase due to PEG addition was observed in case of SP willow (p = 0.022), SP hemp (p = 0.032), SP spruce (Cel) (p = 0.002) and SP spruce (Cel + Nov) (p = 0.019), while there was absolutely no effect of PEG addition in case of SP corn stover (p = 0.228), SP wheat straw (p = 0.860) and SP sweet sorghum bagasse (p = 0.120).
3.3 Protein adsorption during hydrolysis
Protein concentrations measured in the 72-hour hydrolysis supernatants are presented on Fig. 1. Significant increase in protein concentration in the hydrolysis supernatant in case of SP spruce (Cel + Nov) (p = 0.0002), SP spruce (Cel) (p = 0.0002), SP hemp (p = 0.002), SP willow (p = 0.00005) and SP wheat straw (p = 0.002) was observed. For SP corn stover the difference between the ‘with PEG’ and ‘without PEG’ cases was not significant (p = 0.156), while for SP sweet sorghum bagasse a significant (p = 0.002) but reversal trend was observed. The most pronounced increase in protein concentration due to PEG addition was observed for SP spruce (both SP spruce (Cel) and SP spruce (Cel + Nov)). The decrease in protein concentration can be regarded as adsorption.
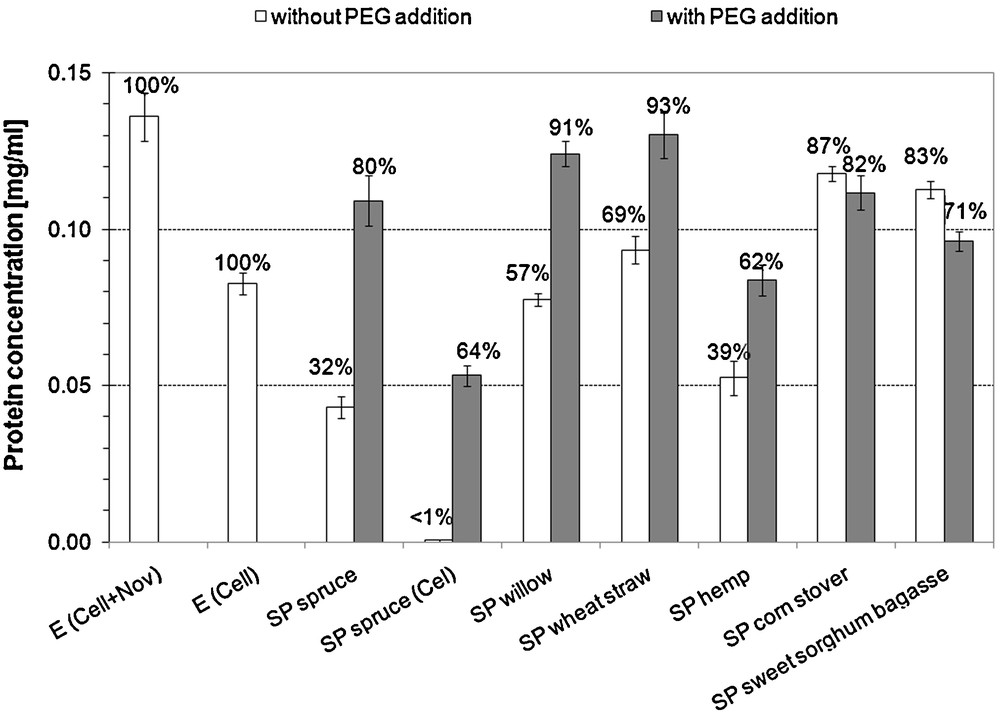
Protein concentrations in the 72-hour hydrolysis supernatants. The ratio compared to the originally loaded is indicated in the top of each column (0.083 and 0.136 mg/mL protein was initially loaded for the one-enzyme (Cel) and the two-enzyme (Cel + Nov) system, respectively). E: enzyme blank. For SP spruce Cel: only Celluclast 1.5L loaded, Cel + Nov: Celluclast supplemented with Novozym 188. For all other substrates, the two-enzyme system was applied.
3.4 Free enzyme activities in the hydrolysis supernatants
The free FP and β-glucosidase activities at the end of the 72-hour hydrolysis have been measured (Fig. 2A and B). In case of SP spruce (Cel + Nov) about 90% of the initial FPA have disappeared from the hydrolysis supernatant, while in case of other substrates, the decrease of enzyme activity was less pronounced, resulting in 50–60% decrease. In the case of SP spruce (Cel), the decrease of FPA in the hydrolysis supernatant was remarkably higher than for SP spruce (Cel + Nov). For all substrates, decrease of FPA in supernatant was reduced by PEG addition. The highest effect of PEG addition was in case of SP spruce (7.7-fold higher activity for SP spruce (Cel + Nov) and about 90-fold increase for SP spruce (Cel)), while no significant difference (p = 0.571) due to PEG addition was observed on SP sweet sorghum bagasse. For SP corn stover, SP hemp, SP willow and SP wheat straw, 53%, 64%, 68% and 51% increase in FPA was observed, and the difference was found to be significant (p < 0.0015).
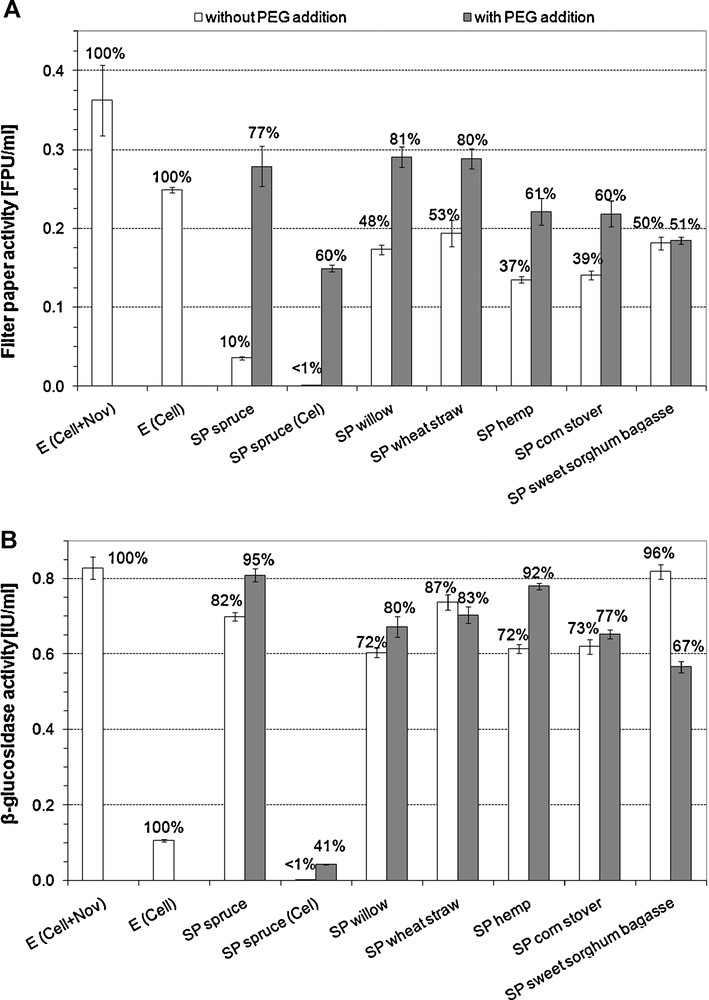
FP (A) and β-glucosidase (B) activities in the hydrolysis supernatants. The ratio compared to the originally loaded activity is indicated on the top of each column. E: enzyme blank. For SP spruce Cel: only Celluclast 1.5L loaded, Cel + Nov: Celluclast 1.5L supplemented with Novozym 188. For all other substrates, the two-enzyme system was applied.
For β-glucosidase, the decrease of enzyme activity and the difference between the ‘without PEG’ and the ‘with PEG’ cases were less pronounced when both Celluclast 1.5L and Novozym 188 were used. The positive effect of PEG addition on the free β-glucosidase activity was significant for SP spruce (Cel + Nov) (p = 7 × 10−4), SP spruce (Cel) (p = 10−5), SP willow (p = 0.017) and SP hemp (p = 4 × 10−5), while the increase has not reached the level of significance with SP corn stover (p = 0.063). Reverse effect of PEG addition on free β-glucosidase activity in case of SP sweet sorghum bagasse (p = 4 × 10−5) and SP wheat straw (p = 0.117) could be noticed. The most pronounced difference was observed in hydrolysis of SP spruce with Celluclast 1.5L alone, where a 100-fold increase in free β-glucosidase activity was observed due to the addition of PEG.
3.5 Pyrolysis-GC/MS analysis of steam pretreated substrates
The steam pretreated substrates were analysed for the characteristics of the lignin structure by Py-GC/MS. When the pyrolysis parameters are set carefully, mostly the ether bonds between the monomeric units are disrupted during pyrolysis and monomeric lignin products are released. However, other chemical bonds are also affected; mainly the aliphatic side chains are cleaved. The main groups of lignin pyrolysis products are visualised in Fig. 3. SP spruce, the substrate with the highest affinity for adsorbing enzymes has differed regarding the ratio of pyrolysis products compared to the other substrates (Table 4). It released the highest yield of dihydroxybenzenes, although the original spruce lignin contained mostly 2-methoxyphenol segments (data not shown). This can be explained by the demethylation of the aromatic methoxyl-groups during the steam pretreatment which is in good accordance with previous reports [44,45]. Relatively high ratio of pyrolysis products with carbonyl groups on the alkyl side chain were also produced from SP spruce together with SP willow and SP hemp. On the other hand, only small amount of alkenyl phenols and H-lignins (no methoxyl substitutions) are formed from the wood samples (SP spruce and SP willow) and these products are, as expected, more abundant from the other plant samples.

Groups of lignin pyrolysis products.
Ratio of lignin derived pyrolysis products for various steam pretreated substrates. The phenolic compounds were measured by GC/MS.
Carbonyl compounds | Dihydroxy-benzene compounds | Vinyl compounds | Alkenyl compounds | H-lignin | |
Pyrolysis product/total lignin products | |||||
SP spruce | 0.154 | 0.273 | 0.100 | 0.158 | 0.152 |
SP willow | 0.171 | 0.123 | 0.168 | 0.225 | 0.159 |
SP wheat straw | 0.124 | 0.110 | 0.225 | 0.283 | 0.200 |
SP hemp | 0.173 | 0.030 | 0.288 | 0.430 | 0.151 |
SP corn stover | 0.052 | 0.034 | 0.441 | 0.506 | 0.510 |
SP sweet sorghum bagasse | 0.065 | 0.022 | 0.388 | 0.467 | 0.360 |
3.6 Quantification of hydroxyl groups and carboxylic acid moieties in lignin using 31P NMR
In order to clarify the chemical modifications occurred in lignins before and after the hydrolysis processes, we used an advanced heteronuclear magnetic resonance analysis, the 31P NMR. This technique provides quantitative and selective information of labile protons on hydroxyl groups present in lignins, namely the aliphatic OH groups, the different phenolic OH and carboxylic acids after the sample in situ phosphytilation [46,47]. In particular, the samples were phosphitylated with 2-chloro-4,4’,5,5’-tetramethyl-1,3,2-dioxaphospholane in pyridine/deuterated chloroform mixture (1.6:1.0, v/v ratio) with a suitable internal standard and then subjected to 31P NMR analysis [41,42,46,47]. On Fig. 4, spectra of the steam pretreated materials are shown, together with the detailed signal assignment and in Table 5 the quantitative data, for the functional groups, before and after the hydrolysis, obtained by phosphorus NMR are reported. As steam pretreated samples contained significant amount of cellulose and some hemicellulose besides lignin, a factor was calculated from the carbohydrate conversions for the hydrolysis residue samples. Multiplying the results obtained by NMR shows the quantity of various hydroxyl groups present in hydrolysis mixture. The amount of aliphatic hydroxyl groups showed large variations, while most of the substrates contained between 1 and 2 mmol/g SP wheat straw and SP corn stover contained significantly higher and lower amount, 3.05 mmol/g and 0.52 mmol/g respectively. This is probably connected with the pretreatment conditions; for most of the substrates sulphur-dioxide catalyst was used during pretreatment, where for wheat straw, sulphuric acid was supplied. In the corn stover pretreatment no catalyst was added. On the basis of guaiacylic OH groups content; the softwood steam pretreated samples could be divided in two different groups: SP willow and SP wheat straw with an average content of 0.15 mmol/g of this functional group, and SP hemp, SP corn stover, and SP sweet sorghum bagasse, with an average amount more than two times lower (0.06 mmol/g). In the case of condensed hydroxyl groups, SP willow and SP wheat straw contained significantly higher amount compared (average amount 0.42 mmol/g) to the other substrates (average amount 0.1 mmol/g).
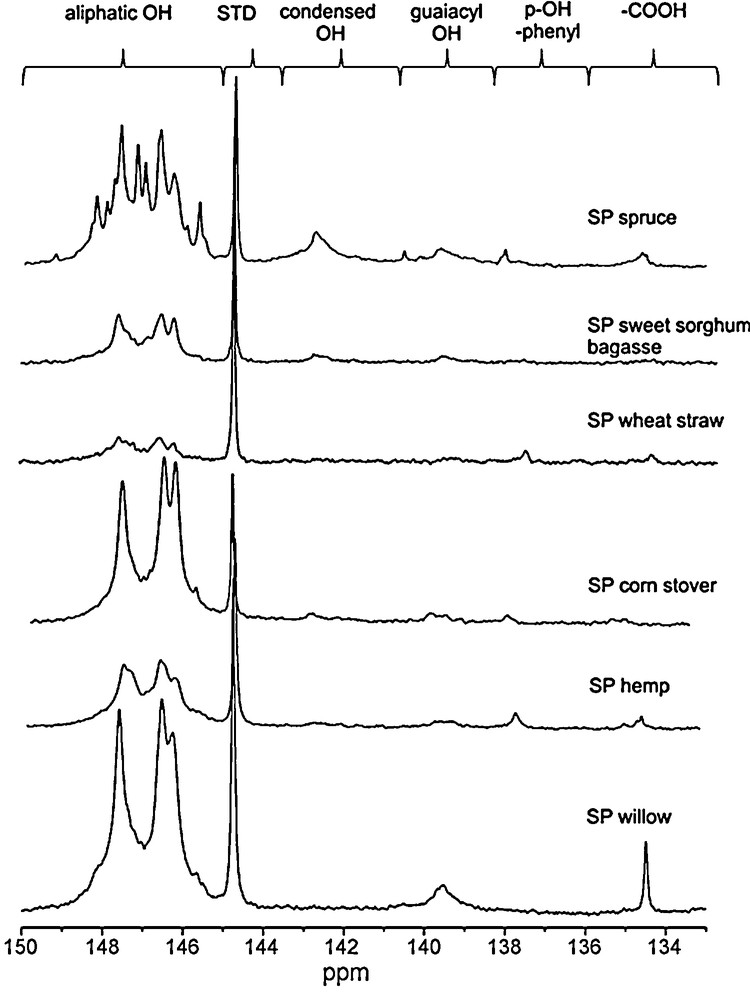
31P NMR spectra of steam pretreated substrates, with the chemical shifts related to the various structures.
31P NMR analysis of the original steam pretreated substrates and that of the hydrolysis residues.
Relative quantity in hydrolysisa | Aliphatic OH | Condensed/siryngyl OH | Guaiacylic | p OH phenyl | Carboxylic | ||
mmol/g solid | |||||||
SP spruce | Steam pretreated material | 1.000 | 1.760 | 0.086 | 0.116 | 0.003 | 0.079 |
Hydrolysis residue | 0.482 | 2.173 | 0.296 | 0.258 | 0.072 | 0.195 | |
Hydrolysis + PEG residue | 0.453 | 2.584 | 0.462 | 0.417 | 0.143 | 0.173 | |
SP willow | Steam pretreated material | 1.000 | 1.957 | 0.428 | 0.158 | 0.079 | 0.194 |
Hydrolysis residue | 0.371 | 2.925 | 0.493 | 0.162 | 0.068 | 0.133 | |
Hydrolysis + PEG residue | 0.341 | 3.258 | 0.731 | 0.257 | 0.127 | 0.180 | |
SP wheat straw | Steam pretreated material | 1.000 | 3.050 | 0.421 | 0.173 | 0.113 | 0.127 |
Hydrolysis residue | 0.371 | 3.931 | 0.473 | 0.274 | 0.187 | 0.234 | |
Hydrolysis + PEG residue | 0.373 | 2.748 | 0.465 | 0.277 | 0.176 | 0.222 | |
SP hemp | Steam pretreated material | 1.000 | 1.130 | 0.132 | 0.072 | 0.028 | 0.098 |
Hydrolysis residue | 0.381 | 4.350 | 0.280 | 0.122 | 0.071 | 0.251 | |
Hydrolysis + PEG residue | 0.356 | 4.339 | 0.290 | 0.145 | 0.079 | 0.162 | |
SP corn stover | Steam pretreated material | 1.000 | 0.516 | 0.078 | 0.042 | 0.055 | 0.083 |
Hydrolysis residue | 0.498 | 1.689 | 0.124 | 0.053 | 0.081 | 0.114 | |
Hydrolysis + PEG residue | 0.518 | 2.949 | 0.384 | 0.198 | 0.172 | 0.329 | |
SP sweet sorghum bagasse | Steam pretreated material | 1.000 | 1.264 | 0.103 | 0.066 | 0.058 | 0.117 |
Hydrolysis residue | 0.471 | 2.626 | 0.274 | 0.149 | 0.184 | 0.243 | |
Hydrolysis + PEG residue | 0.454 | 2.753 | 0.218 | 0.107 | 0.134 | 0.219 |
a Calculated from the carbohydrate conversions.
After the hydrolysis, both with and without PEG addition, the residual lignins showed an increase of aliphatic, condensed and guaiacyl OH groups with respect to the preatreated material. As a general trend the increase in aliphatic OH groups could be explained as the occurrence of hydrolysis reactions of linkages in the lignin side chains, and alternatively to the hydrolysis of lignin-carbohydrate complexes. Moreover, the increase in the amount of guaiacyl OH groups usually suggests the occurrence of alkyl-phenyl ether bond cleavage reactions which finally result in lignin depolymerisation, while the increase of condensed phenolic OH groups is due to the occurrence of oxidative coupling processes on phenolic end groups. However, under the reaction conditions used no oxidation reactions are likely to occur unless the enzymes used were contaminated with traces of oxidative enzymes. Such modifications in lignin structure might be due to hydrolysis of ester bonds throughout the lignin backbone. This finding would explain the relative high amount of carbonyl groups detected by Py-GC-MS.
4 Discussion
4.1 The effect of PEG addition on lignocellulose conversion
The positive effect of addition of surfactants [15,18,19,21,24,25], polymers [16,26,27] and proteins [14] on the enzymatic conversion of pretreated lignocelluloses has already been reported to various extents. The degree of the conversion enhancement depends on several parameters, such as the nature of the substrate [21,48], the used pretreatment technique, the temperature of hydrolysis [16] or enzyme loading [22,23]. In our experiments, the conversion increasing effect of PEG was not pronounced, as relatively high enzyme loading was applied due to the planned adsorption measurements and low (2% DM) substrate concentration because of mass transfer considerations. The different degree of conversions among various substrates is probably determined by the recalcitrance of the substrate or the pretreatment efficiency. When SP spruce was hydrolysed using Celluclast alone or with combination of Novozym 188 (β-glucosidase supplementation), the addition of either PEG 4000 or Novozym 188 resulted the same order of magnitude increase in cellulose conversion (60% for SP spruce (Cel), 77% for SP spruce (Cel) + PEG, 79% for SP spruce (Cel + Nov)), therefore regarding the cellulose conversion, β-glucosidase supplementation and PEG addition alone had an effect with similar magnitude. When no β-glucosidase supplementation was applied, significant amount of cellobiose was also present in the hydrolysis products, which counted for an additional 15% and 9% cellulose conversion for SP spruce (Cel) and SP spruce (Cel) + PEG, respectively. The combination of PEG and Novozym 188 has resulted 84% conversion (SP spruce (Cel + Nov) + PEG).
4.2 Unproductive binding of cellulases during hydrolysis and its prevention with PEG addition
Free enzyme activities tend to decrease during enzymatic hydrolysis [12,27,29]. This comes from: (1) the productive binding of cellulases and occurs in the early stage of hydrolysis, when cellulose is still present [12,27,29]; and (2) from the unproductive binding of cellulases on the substrate, especially on lignin [16,25–27]. We have found that due to PEG addition, the protein adsorption has decreased, and the free enzyme activities, especially for FPA, have increased to different extent using various steam-pretreated substrates. The decrease in protein concentration can be regarded as adsorption, in contrast to the results achieved with free enzyme activity measurements, where other factors, like enzyme inactivation or synergistic effects may also occur. The effect of PEG addition on free protein concentration and free FPA showed a significant correlation (r = 0.868), suggesting that the most of the protein detected was involved in cellulose degradation. The finding that the extent of the effect of PEG addition on various substrates is different is in good accordance with the observation of Kumar and Wyman [48], who have investigated the effect of additives on the hydrolysis of corn stover pretreated using various techniques. However, it should be noted that various pretreatment methods modify the structure of the substrate, especially the structure of lignin differently. During steam pretreatment, lignin undergoes several structural changes, such as: (1) melting and reprecipitating, resulting in dense lignin-agglomerates [49]; (2) degradation and repolymerisation [50]; and (3) other side-chain reactions [50]. As decrease of free FPA was found to be different using various steam pretreated substrates, and similar treatment (acid catalysed steam pretreatment) was applied for the substrates, there might be difference in the lignin structures of the substrates even though the same kind of pretreatment has been used. These lignin structures tend to: (1) bind cellulases; and (2) adsorb PEG to a different extent. The results show that the positive effect of PEG addition is not universal, since there were substrates, like SP sweet sorghum bagasse or SP corn stover, where no positive effect could be observed, while it was pronounced in case of SP spruce. This could be due to the typical softwood lignin structure of spruce that does not show the occurrence of p-hydroxyl and condensed phenolic OH groups, while hardwood lignins contain syringyl units and annual plant lignins contain also significative amounts of p-hydroxyphenyl units.
4.3 Role of enzyme source on β-glucosidase adsorption
There are contradictious results in the literature about β-glucosidase adsorption. Several papers reported significant β-glucosidase adsorption on lignocelluloses [14,51], while others observed less binding of β-glucosidases [12,29,30], latter are more comparable with our previous result [27] obtained with SP spruce (Cel + Nov). The main difference in the referred papers was the origin of the enzymes used in the hydrolysis/adsorption experiments. To clarify this problem, hydrolysis of SP spruce has also been performed with Trichoderma reesei (anamorph of Hypocrea jecorina) enzymes alone (SP spruce (Cel)), i.e. without Aspergillus niger β-glucosidase supplementation. Surprisingly, when only T. reesei β-glucosidase (Celluclast 1.5L only) was present in the hydrolysis mixture almost no free β-glucosidase activity was observed after 72-hour hydrolysis; compared to the other case (SP spruce (Cel + Nov)), where A. niger β-glucosidase (Novozym 188) was also present. This drastic decrease in β-glucosidase activity has been reflected in the decrease of FPA as well. Addition of PEG 4000 has increased efficiently the free β-glucosidase activity in hydrolysis supernatant when only T. reesei enzyme was present, and had less impact on the β-glucosidase activity of the supernatant in case of the Celluclast 1.5L + Novozym 188 mixture. The difference in the adsorption behaviour of β-glucosidases from different enzyme sources may be due to their molecular properties. These enzymes lack carbohydrate binding modules, which were previously shown to be important in unproductive binding of cellulases [16]. Other surface properties affecting the enzyme adsorption on lignin, like exposed hydrophobic amino-acid side chains or different degree in protein glycosylation could also be the reason for this difference.
4.4 Structures of lignins in various steam pretreated lignocelluloses
Lignin is a three-dimensional cross-linked polyphenyl structure built up of 4-hydroxyphenylpropanoids that differ in their degree of methoxylation; (1) p-coumaryl alcohol (no methoxyl group); (2) coniferyl alcohol/guaiacyl (one methoxyl group in position 3) and (3) sinapyl alcohol/syringyl (two methoxyl groups in position 3 and 5) [52]. The linkages (carbon-carbon and ether bonds) between the units are mostly formed between the p-hydroxyl groups and/or the propyl-side chain substitutions.
The analysis of pyrolysis products of the various steam pretreated substrates has shown that the thermal degradation of the lignins was different, suggesting that the structure of the steam-pretreated lignins were different too. The distribution of the phenolic pyrolysis products in case of SP spruce was significantly different from the other five substrates, which is in accordance with the results obtained with the amount of unproductively bound FPA or protein adsorption. Correlation analysis between the pyrolysis products and the positive effect of PEG addition on the free FPA (percent increase in free FPA due to PEG addition) has shown a significant correlation (r = 0.94) with the ratio of the dihydroxybenzene compounds. The presence of dihydroxybenzene compounds among the pyrolysis products suggests the presence of free (not methylated) phenolic hydroxyl groups in the steam-pretreated lignins indicating the removal of methyl groups from the methoxyl groups during the pretreatment.
The higher amount of dihydroxybenzene products in case of spruce is probably due to the fact that softwood lignin mostly built up from guaiacyl subunits, while hardwood lignins consist of guaiacyl and syringyl subunits. Lignins of grasses and perennial plants are built up from guaiacyl, syringyl and p-hydroxyphenyl subunits.
Quantitative analysis of functional groups of lignins with 31P-NMR, before and after the hydrolysis processes, has shown less variability of the samples. The total phenolic content was comparable in the different substrates, except for SP willow and SP wheat straw, which showed a higher value. These two substrates together with SP spruce had remarkably higher guaiacyl hydroxyl content. Notably PEG addition was the most effective in decreasing protein adsorption in case of these substrates; however, SP spruce was not as exceptional as with the Py-GC/MS analysis. However, with the NMR analysis, no differentiation between demethylated guaiacyl groups and ether-bond disrupted guaiacyl structure with the methyl group can be made. After the enzymatic hydrolysis, an increase of the total phenolic content occurred that was more evident in SP spruce and SP sweet sorghum bagasse without PEG and in SP corn stover with PEG addition.
4.5 Mechanism of PEG effect on enzyme adsorption
Addition of PEG in enzymatic hydrolysis of steam pretreated substrates was shown to increase free cellulase activity in the hydrolysis supernatant. PEG has been previously found to adsorb on the substrate in enzymatic hydrolysis [26] and when incubated with SP spruce alone [16]. In addition, insignificant PEG adsorption was observed on microcrystalline cellulose [16], suggesting that PEG adsorbs on the surface of the lignin. Our experiments have shown that the amount of dihydroxybenzene pyrolysis products correlated with the increase of free FPA due to PEG addition.
The interactions occurring in protein adsorption have been recognised as non-covalent interactions such as: (1) hydrogen bonding; (2) electrostatic; or (3) hydrophobic interactions [53]. It has been shown that surfaces with polyethylene oxide grafts show greatly reduced protein adsorption [54]. PEG can attach to the lignin: (1) terminally; or (2) by interaction between the lignin and an internal atom of the polymer. The first interaction results in a ‘fur-like’ coat on the lignin surface with high volume excluded by PEG and require high PEG dosage to cover the whole surface, while the latter results in a thin PEG layer on the surface and require less PEG.
Our results suggest that the interaction is formed between PEG and the phenolic hydroxyl groups of lignin. The importance of phenolic hydroxyl groups in lignin and cellulase inactivation has already been hypothesised [28]. Lignin modified by hydroxypropylation (specific modification of phenolic hydroxyl group) was found to be less detrimental to cellulose hydrolysis, than unmodified lignin [28]. Phenolic hydroxyl groups exposed on the lignin surface can easily form hydrogen bonds with the internal oxygen atoms of the PEG polymer. This would mean that in this case PEG is accepting, and free phenolic hydroxyl group of lignin is donating the hydrogen bond. It has already been suggested that ethylene oxide based surfactants are hydrogen bond accepting ones [16]. However, the occurrence of hydrophobic interaction cannot be excluded with our experimental results.
5 Conclusion
Addition of PEG polymer to enzymatic hydrolysis of steam pretreated lignocelluloses was found to be beneficial by increasing the free cellulase activity in the hydrolysis supernatant. The increased concentration of active cellulases can result in a faster and more efficient cellulose conversion, a possible decrease in enzyme need or possibility for enzyme recycling.
The positive effect of PEG addition was found to be different using various pretreated substrates, with steam pretreated spruce showing the highest affinity for enzymes. The results suggest that the different degree of increased free cellulase activity obtained by PEG addition is based on the various structures of lignin in the substrates. It was concluded that phenolic hydroxyl groups exposed on the lignin surface interacts with PEG through hydrogen bonding, forming a layer of PEG on lignin surface, which prevents unproductive binding of cellulases on lignin.
Decrease of free β-glucosidase activity was found to be dependent on the source of the enzyme. T. reesei β-glucosidase (in Celluclast 1.5L) tends to bind unproductively in higher degree than A. niger β-glucosidase (in Novozym 188). Our results suggests that increasing β-glucosidase secretion of T. reesei in the enzyme fermentation is not the key solution for the problem of β-glucosidase deficiency of the culture broths, but addition of β-glucosidase from other enzyme sources may be more beneficial.
Disclosure of interest
The authors declare that they have no conflicts of interest concerning this article.
Acknowledgements
This work was supported by the framework of the European Cooperation in the field of Scientific and Technical Research (COST Action FP0602, Biotechnology for Lignocellulose Biorefineries). This work was financially supported by the Hungarian National Research Fund (OTKA – K 72710 and K 81959). This work is connected to the scientific program of the “Development of quality-oriented and harmonized R + D + I strategy and functional model at BME” project, supported by the New Hungary Development Plan (Project ID: TÁMOP-4.2.1/B-09/1/KMR-2010-0002). Francesco Zimbardi (ENEA, Italy) and Prof. Guido Zacchi (Lund University, Dept. of Chemical Engineering) are gratefully acknowledged for the steam pretreated substrates. Prof. Sándor Kemény (Dept. of Chemical and Environmental Process Engineering, Budapest University of Technology and Economics) is kindly acknowledged for the valuable discussions about statistical analysis.