1 Introduction
Although the rechargeable lithium-ion battery, first introduced in 1990, has been the most important advance in energy storage in 100 years, it falls short of meeting technological demands. Further breakthroughs are sorely needed. Aware of such a great challenge, numerous chemists have successfully acted at several levels to incrementally improve the performance of present Li-ion systems. However, it is becoming apparent to all of us that we are progressively reaching the limits in terms of performances. When such a situation arises in science, it is important to look for new directions and open new avenues.
Surprisingly, although nanomaterials, nanostructured components are at the origin of today’s staggering micro-electronic progress, chemists have mainly persisted in following a macroscopic approach in the search for electrode materials. Luckily, over the last years, a few groups have deviated from the usual path to tackle nanostructured electrode materials and experience encouraging trends and fascinating new effects. Among them, our group who decided to revisit several classes of materials precluded to react with lithium under the classical insertion/deinsertion mechanism. Through such a materials survey, totally unexpected results were brought on the scene. Among them are (1) the reactivity of the interstitial-free metal oxides structure towards lithium [1–5], through conversion reactions, leading to sustained reversible capacities ranging from 700 to 900 mA h g–1, and entailing in situ novel polymerization/depolymerization reactions as it will be discussed, (2) the possibility of radically changing reaction kinetics and chemical/electrochemical reaction paths of inorganic materials vs Li by going from macro- to nanoparticles as observed with Co3O4 [6] or α-Fe2O3 [7], and (3) the discovery of reversible copper extrusion–insertion electrodes, such as Cu2.33V4O11 [8], for rechargeable batteries that operates through displacement reactions similar to those previously reported by Thackeray et al. [9,10] for intermetallic alloys such as Cu6Sn5 or Cu2Sb [11].
So, as we move from bulk materials towards systems with small particles, new electrochemical phenomena are observed, and we will describe the fundamentals underlying such new phenomena of conversion vs displacement reactions, the new opportunities that they offer with respect to energy storage in general, while also stressing the obstacles that must still be overcome to turn such new concepts into practical batteries.
2 Results and discussions
2.1 Conversion reactions
In the search for insertion electrodes, 3d-metal oxide compounds having empty channels or layered materials have been intensely investigated with the discoveries of highly performing compounds such as LiCoO2, LiMn2O4 or LiFePO4 that can, at most, exchange one electron per 3d-metal. In contrast, binary oxides having rocksalt-type structures and thus no free voids to host Li were disregarded for such applications. Therefore, in studying the electrochemical activity of Co-based vanadate compounds [1] towards Li, we observed a capacity greater than expected, assuming the full reduction of the metal cations. Searching for the origin of this extra capacity, we ended up testing the electrochemical performance of CoO vs Li and, to our surprise, we observed a reversible two-step reduction process (Fig. 1). Through an arsenal of characterization techniques involving X-rays, HREM, XANES, Mössbauer and electrochemical techniques, we could deduce that such reduction reaction was first entailing the decomposition of CoO into 20- to 30-Å Co nanoparticles embedded into an Li2O matrix (Fig. 1, inset) on the 0.8-V plateau, and then the formation of a veil-like polymeric layer beyond x = 2 when the voltage smoothly and continuously decreased down to 0.01 V. Owing to the nanometric/composite nature of the so-obtained electrodes, these conversion reactions (Co2+ ⇌ Co) were shown, for the first time, to be highly reversible, providing large capacities (740 mA h g–1) that can be maintained for hundreds of cycles at room temperature.

Voltage composition curve for a CoO/Li cell cycled between 0.01 and 3 V with, as an inset, a TEM micrograph showing the nanostructured composite electrode at the end of the discharge process.
Similarly, the low-voltage capacity, associated to the appearance/disappearance of a polymer-like film around the metal nanoparticles, was found to be reversible [12]. The formation of this film was supposed to be rooted in an electrochemically-driven electrolyte degradation mechanism catalysed by the presence of nanoparticles. Through an arsenal of characterization tools enlisting IR and mass spectrometry, the gel-like organic film formed during the reduction of CoO/LiPF6/ Li was identified as being mainly made of phosphate-ending PEG-(polyethylene glycol)-type chains. Such a polymeric layer was found to be beneficial to the cell cyclability, because it acts as an envelope holding the nanoparticles together. However, in spite of all the studies so far undertaken, we are still debating between solvated Li-ions, electroactive organic molecules, and Li under potential deposition as the possible origin of such an observed low capacity. We experienced [13] that such extra capacity was positively affected by the temperature (doubling of the capacity at 75 °C), and furthermore showed that CoO/Li cells could nicely sustain their capacity over 600 cycles (Fig. 2) at high rates without any dendrite formation, while it is well established that high rates are keen conditions to favour dendrites. Hence the beneficial role that such an organic layer could play in fighting dendrite formations. Such a similar temperature-driven capacity enhancement was also observed by Amatucci et al. [14] on Cu3N/Li cells, implying that such an effect was neither specific to metal oxides nor to the nature of the 3d-metal involved, but more to the electrodes containing dispersed metal nanoparticles.
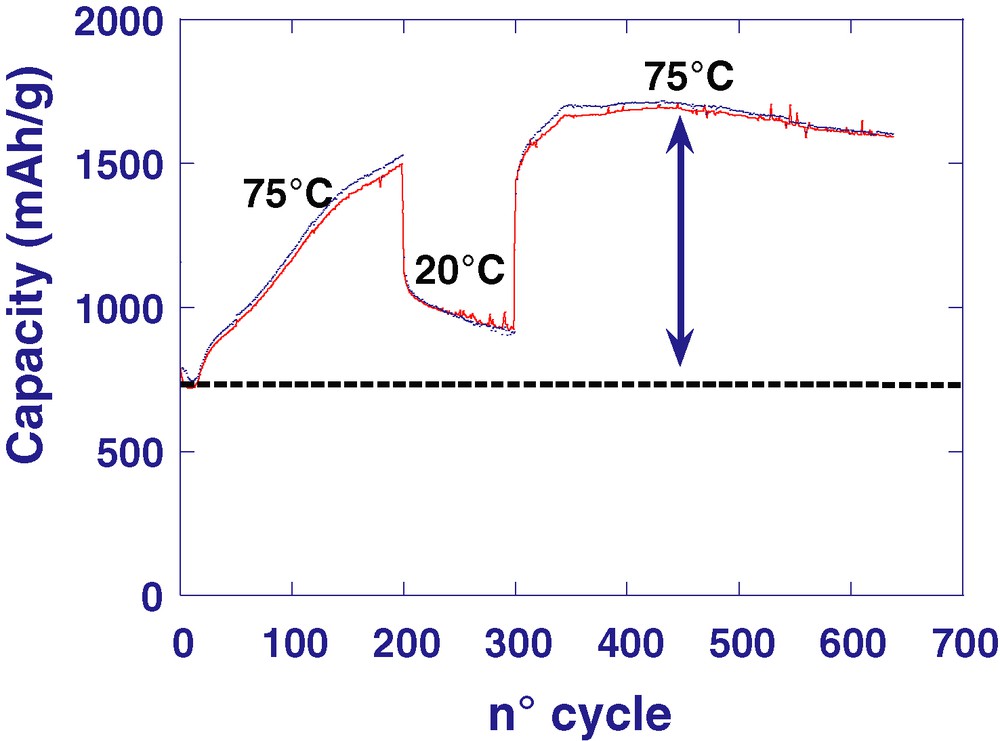
Capacity vs cycle number for a CoO/Li cell cycled between 0.01 and 3 V at a temperature of 75 °C. Note that the capacity returns to its initial value when the cycling temperature is lowered down to 20 °C.
Soon after such a pioneering work, many other sightings of conversion reactions processes in either nitrides, fluorides, phosphides, sulphites, and borates were reported and shown to involve, depending on the oxidation state of the 3d-metal, 1 (Cu2O), 2 (CoO), 3 (Fe2O3) or 4 (RuO2) electrons per 3d-metal, thus offering the possibility of having compounds based on low-cost elements such as Mn and Fe, with staggering capacity gains over the existing ones. Besides, by playing with the electro-negativity of the anion, one can tune the potential of the conversion reactions and achieve large V values as we ‘marched’ from oxides to fluorides, for instance. Along that line, fluoride-type compounds such as FeF3 [15] and CoF3, showing a conversion potential of about 2.5 V and having Fe and Co in its +3 oxidation state, are quite appealing electrode materials, since they could theoretically give energy densities as high as 800 mW h g–1 of material. Besides, one should realize that the appeal of conversion reactions involving oxides, nitrides, fluorides lies also in the nanostructured character of the electrode that is internally created (Fig. 3) during the first electrochemical reduction, producing pristine and compact nanoparticles, which are held together through LinX (X = O or F) boundaries domains so that low-packing density issues associated to the use of externally-made nanostructured powder do not exist.
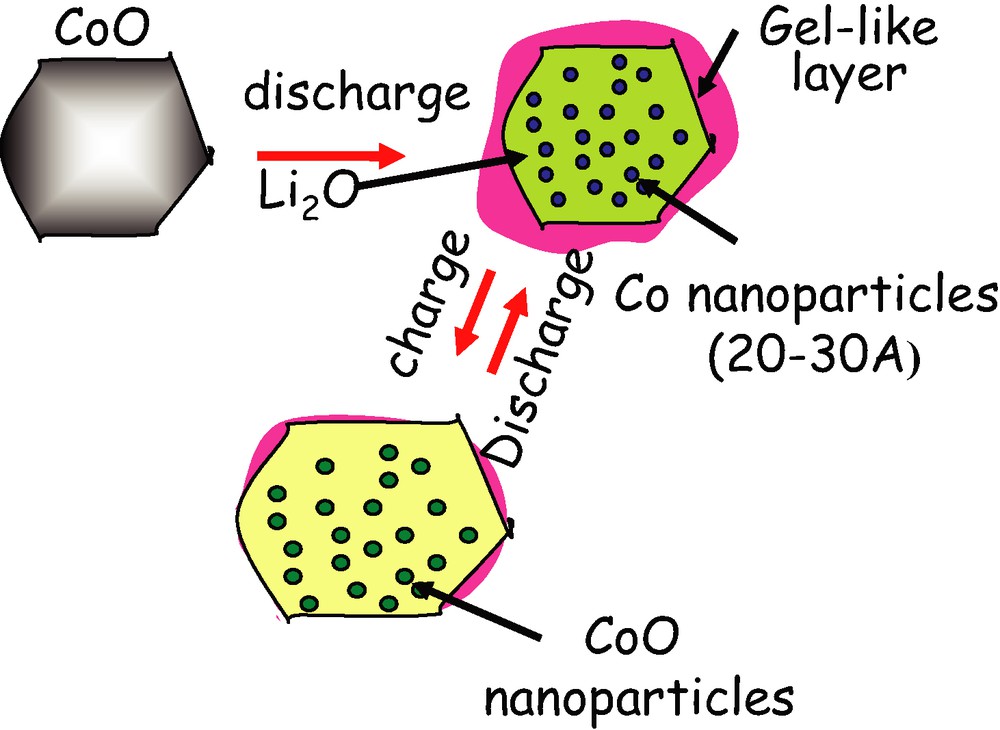
Schematic of the conversion reaction process showing both the formation of Co nanoparticles and of the gel-like polymeric layer as well as their evolution upon subsequent charges/discharges.
However, such excitement should be tempered due to the fact that the kinetics of such reversible conversion reactions (e.g., the rate at which the electro-active species together with electrons can reach the interfacial region where Li reacts with the active domains) are poor. Thus, their future with respect to their applications lies in mastering their kinetics. While great progresses have being achieved, further intense research remains to be done on those conversion reactions, as expected with any new concept. Therefore, this obstacle is not insurmountable, and there are possible solutions. The first to come to mind, and already proven to work, is the temperature with the best example being the development, a few years ago, of ZEBRA-type cells, based on the (Ni2+Cl2 + 2 Na+ + 2 e– ⇌ 2 NaCl + Ni) conversion reaction, which operates at 400 °C. One believes that similar kinetics should be achievable with an Li electrochemically-driven conversion reaction at room temperature by both using nanoscale electrochemistry and acting on the nanoparticle surface either at the engineering level, by dispersing metal nanopowders into nano-oxides/nanofluoride powders, or using nanofibril supporting textures (such as carbon) to host our nanosized powders, or at the chemical level by extending the carbon ‘nanopainting’ route, so successful with LiFePO4, to nanopowders oxides, nitrides or fluorides. In summary, such conversion reactions that mainly boil-down to an Li-driven electrochemical decomposition of the material into a nanostructured electrode totally differs from the second new concept that we are presenting next, and for which there is preservation of a structural relationship between the precursor and the cycled phase.
2.2 Displacement reactions
As for conversion reactions the concept of displacement reactions is not totally brand new, since a few reports back in the 1980s were a sign of Li-electrochemical driven metal extrusion process on Cu-based compounds. Nevertheless, the interest in such reaction was revived three to four years ago by Thackeray’s work [9,10] on intermetallic alloys. The authors show that intermetallic phases such as Cu6Sn5 and Cu2Sb that, show a strong structural relationship to their lithiated products, Li2CuSn and Li3Sb are quite attractive as electrode materials, respectively. Indeed, they operate through a mechanism involving a reversible lithium insertion/metal extrusion process with an invariant face-centred cubic Sb array, therefore minimizing the alloy overall expansion, and thus facilitating their possible use as negative electrode materials. Aware of such an elegant approach, we decided to explore this concept to design new positive electrode materials. Searching for potential candidates for such reactions we revisited the n CuO–V2O5 [16,17] phases, some of which were previously reported to have shown partial reversible copper extrusion or some of the Ag-based vanadate phases such as Ag2V4O11 [18], which has been intensively studied in the past, since such a phase is presently used as the active electrode material in primary power sources for implantable cardio defibrillators. Going through such a survey, we could identify a new phase, Cu2.33V4O11, that electrochemically reacts with Li in a reversible way through a displacement reaction as we will present next.
Cu2.33V4O11 was synthesized as a single phase by reacting at 540 °C under vacuum, in a silica ampoule, stoichiometric amounts of Cu2O, V2O5, and V2O4 oxides. This compound crystallize in a monoclinic structure [19] that can be viewed as a packing of [V4O11]n layers. along the c-axis with their cohesion being provided by copper ions distributed along oxygenated channels running along the b-axis. From both structural refinement and XPS experiments [20,21], the charge-balance distribution between the different cations was sorted out, leading to a material of formula [Cu+1.22]2,3,4[Cu2+1.11]1V5+2.56V4+1.44O11, and the copper site occupancy deduced. It turns out that the divalent copper lies on a slightly distorted tetrahedral site (Cu1), while the monovalent one is distributed over three delocalized sites (Cu2,Cu3,Cu4), which have linear or planar triangular coordination (Fig. 4, inset). Owing to the presence of two redox centres (Cu2+/Cu+) and (V5+/V4+), one could expect such a material to present a rich electrochemistry.

Voltage composition curve for a Cu2.33V4O11/Li cell cycled between 1.5 and 3 V with, as an inset, the crystal structure of the precursor phase.
Swagelok cells, using Cu2.33V4O11 as the positive electrode, Li as the negative electrode, and a Whatman GF/D borosilicate glass fibre sheet saturated with a 1-M LiPF6 electrolyte solution in 1:1 dimethyl carbonate:ethylene carbonate as separator/electrolyte were assembled in a dry box and then tested by starting with reduction or oxidation.
A Cu2.33V4O11/Li cell was first started on discharge and cycled between 1.5 and 3.5 V at a C/5 rate. The voltage–composition curve (Fig. 4) first shows an initial drop of the voltage that nearly reaches x = 0.6, a plateau located at 2.7 V, whose amplitude is about 4 Li, then an extra Li can be reacted as the potential is lowered down to 1.5 V. Although the shape of the recharge curve differs from the discharge one, most of the 5.6 uptake lithium can be removed, leading to reversible capacities ranging from about 250 to 260 mA h per gram of Cu2.33V4O11, that is sustained upon cycling for at least 30–35 cycles [8]. Such results did come as a surprise and were in full contrast with the prevailing view of the last 20 years, since the precursor phase, Cu2.33V4O11, did not contain any empty free sites/channels to host lithium. Thus, the need to get further insights into the unusual reactivity mechanism of Cu2.33V4O11 towards Li. For such a purpose, both in situ X-ray diffraction measurements were performed [8] on a Cu2.33V4O11/Li cell when cycling and HREM measurements were performed on Cu2.33V4O11 electrode materials that were recovered from a Cu2.33V4O11/Li cell that was discharged and charged to various states.
In situ X-ray data (Fig. 5, left), which shows the appearance of the copper main Bragg peaks to the expense of the Cu2.33V4O11 main peaks during the discharge step, suggests a Li-electrochemically driven copper extrusion process associated to a structural collapse of the structure. The overall process was shown to be fully reversible, since the X-ray powder pattern of the fully recharged material was like the one of the starting phase, without any copper Bragg peaks remaining. Such a finding was nicely confirmed by TEM images (Fig. 5, right) that reveal the presence of copper dendrites popping out of an amorphous matrix for the discharged sample and of a well-crystallized material, free from of copper traces, for the fully recharged sample. Overall, this new reaction entails a reversible Li-driven displacement process leading to the growth/disappearance of Cu dendrites with a concomitant reversible decomposition/crystallization of the initial electrode material. We should emphasize that among all the other investigated Cu or Ag-based phases, we did not encounter the same efficiency from the reversible extrusion process. We believe that the uniqueness of Cu2.33V4O11 is nested in the peculiar flexibility of the stacked [V4O11]n layers, owing to the presence of pivot oxygen atoms.

On the top left, the voltage–composition curve is shown for an in situ X-ray Cu2.33V4O11/Li electrochemical cell that was cycled between 1.5 and 3.5 V. Underneath, the X-ray scans taken when the cell was fully charged or discharged are shown. The greenish and red Bragg peaks refer to the Cu2.33V4O11 phase and metallic Cu, respectively. On the right, we have reported in (a) a TEM image realized on the starting electrode material, showing the initial Cu2.33V4O11 platelets together with the corresponding SAED pattern, in (b) a TEM image realized for the fully-reduced electrode showing copper dendrites that are well crystallized as deduced by the corresponding SAED and in (c) a TEM image realized on the re-oxidized electrode up to 3 V, showing that the platelets of Cu2.33V4O11 are re-created as indicated by the corresponding SAED pattern taken on particles lying on different directions than in (a).
Interestingly, when a Cu2.33V4O11/Li cell was started on charge, with an upper cut-off voltage of 4.7 V, we found that we could remove 1.3 electrons, suggesting some copper removal from the compound. This was confirmed by chemical titration of the obtained powder that indicates solely the presence of Cu2+ and V+5, suggesting the feasibility of synthesizing a new phase of formula Cu1.0V4O11 by electrochemical oxidation. Similarly such a new phase could be synthesized by mimicking the electrochemical process by a redox reaction consisting of the oxidation of Cu2.33V4O11 by using a 1-M NO2BF4 acetonitrile solution as oxidizing agent. The structure of this new compound is under determination and its electrochemical behaviour towards Li differs quite strongly from that of the Cu2.33V4O11 phase, as will be reported in an upcoming paper [22,23].
Such a copper extrusion process with namely the appearance/disappearance of a few micron-long dendrites, although quite spectacular, is quite unusual and intriguing. A legitimate question regards the way such filamentary copper grows. A possible scenario is as follows. During cell discharge, the incoming Li ions enter the structure and chase the Cu ions to the particle edge where they are reduced, leading to copper nucleation and copper dendrite growth. On recharge, for the reaction to proceed in the reverse side, one must oxidize copper metal to produce Cu+ or Cu2+ ions in solution, which will rapidly enter the structure. Thus we are dealing, among others, with a Cu/Cu++(Cu+) redox process, which is either occurring at a solid/solid interface (discharge) or at a solid/liquid interface (charge). Such a difference could well be at the origin of the voltage-composition trace differences previously observed between the discharge and charge of a Cu2.33V4O11/Li cell. Finally, while beyond the scope of this paper, another worth-mentioning positive attribute of such Cu-based displacement reactions resides in the electrode conductivity enhancement due to the filamentary copper growth and therefore in the feasibility of designing electrodes with enhanced power-rate performances. Thus, fully reversible displacement reactions could provide a new direction for developing an alternative class of higher energy-density/power-rate electrode materials. However, although the science of reversible Li intrusion/extrusion reactions is fascinating, there are critical issues concerning practical considerations that should not be overlooked, namely copper migration affecting the long-term electrode cyclability.
3 Conclusions
Two new types of reversible Li-driven electrochemical processes involving conversion reactions and displacement reactions were presented respectively. We have reported that electrode materials, operating according to either of the above mechanisms, once optimized, could have enhanced electrochemical performances as compared to today’s electrode materials involving intercalation reactions. With such findings dealing with either electrochemistry at the nanoscale (e.g., conversion reactions) or with Li-driven Cu extrusion/insertion processes, a new path full of opportunities has opened, since in the quest for new electrode materials we no longer need materials with interstitial voids for guest ions or good electronic conductivity. The Li-battery research is entering a new age and an exciting adventure awaits us.
Acknowledgments
The authors are indebted to J. Galy willingness for sharing with us his long-lasting experience on vanadates, as well as D. Larcher and L. Dupont for fruitful discussions.