1 Introduction
The synthesis of new inorganic materials, particularly those which contain organic structural units, has become an extensively pursued research area. These hybrid materials are formed from metal centers that are connected in one, two, or three dimensions with organic linkers (Fig. 1). Interest in such materials, especially those that extend in three dimensions (i.e. metal-organic frameworks [MOFs]), is due to their range of structural topologies and their potential to have tunable properties with important applications in areas such as catalysis [1,2], luminescence [3,4], and sensing [5]. The accurate prediction of products formed in these syntheses however, is not always straightforward; indeed, there are many factors to consider, including metal-organic coordination modes and geometries, metal speciation, and even organic reactivity. To this end, achieving targeted solid-state architectures with some level of predictability is a current focus of many research groups, though the ability to ‘design’ such materials is an open debate especially within the MOF community [1,6].
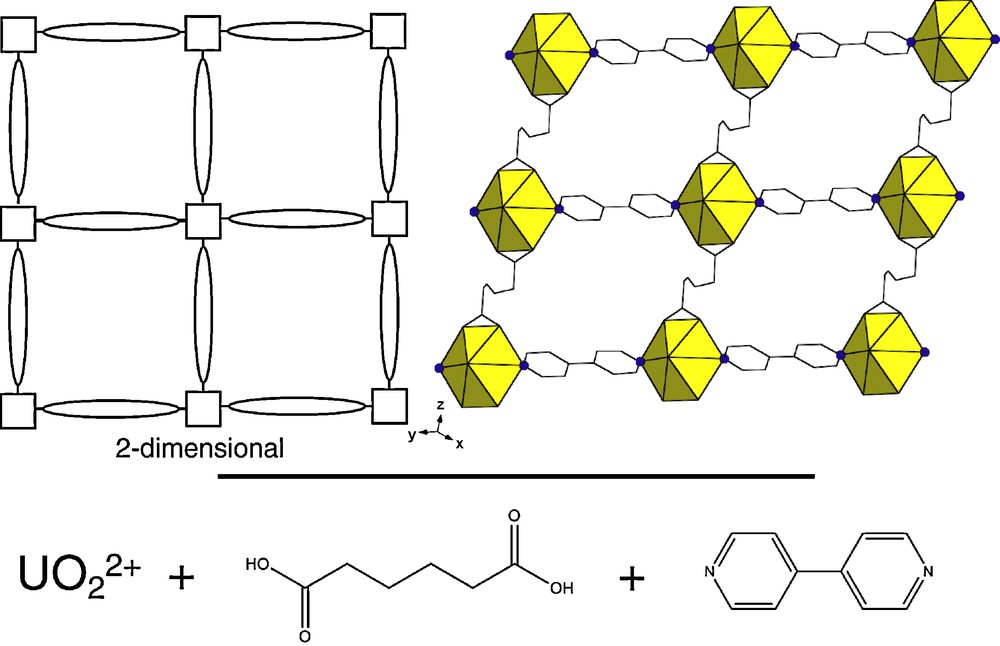
Top left: a skeletal representation of a 2-dimensional hybrid material. The inorganic building units (squares) are connected through organic linker molecules (ovals) to form the extended structure; top right: a 2-dimensional hybrid material consisting of uranium monomeric inorganic building units and two organic linkers (adipic acid and 4,4′-dipyridyl) [12]; bottom: a schematic of the above example. Although a 2-dimensional example is shown, both 1- and 3-D hybrid materials are possible [10].
Crystal engineering generally refers to the ability to control solid-state architectures through the self-assembly of molecular building units, and by extension tuning their chemical and physical properties [7–9]. According to a review by Brammer that highlights developments within inorganic materials engineering, pursuit of controlled architectures has resulted in two distinct classes of materials [8]. The first consists of materials that contain ligands which link metal centers together via metal-ligand coordination. The second class of inorganic-organic materials described are those that result from non-covalent interactions which link the extended networks together. This latter class includes the materials presented herein, and we indeed frame many of our comments in context of Brammer's review.
Looking specifically at hexavalent uranium systems, metal-ligand coordination is a common approach for creating new, diverse structure types [10–15]. One clear advantage to using metal-ligand coordination in the creation of new uranium-organic hybrid materials is that there are many different structure types and architectures seen in the products. However, there are some disadvantages from an engineering perspective when synthetically exploring new materials in this manner. First, accurate prediction of coordination motifs remains difficult, especially for multi-functionalized ligands. Second, there is little control over metal ion speciation in these systems, and uranyl hydrolysis often occurs, resulting in the formation of various secondary building units. These secondary building units are known to include dimers, trimers, tetramers, and hexamers as well as chains and sheets formed from subsequent polymerization. We highlight the diversity, and by extension the unpredictability, of uranium speciation in Fig. 2, noting these particular examples are intended to show a sample that is representative of uranyl speciation in rather simple hybrid systems. Thus, while diverse structure-types have been achieved, prediction of product formation remains difficult within uranyl-organic systems, which employ strictly metal-ligand coordination.
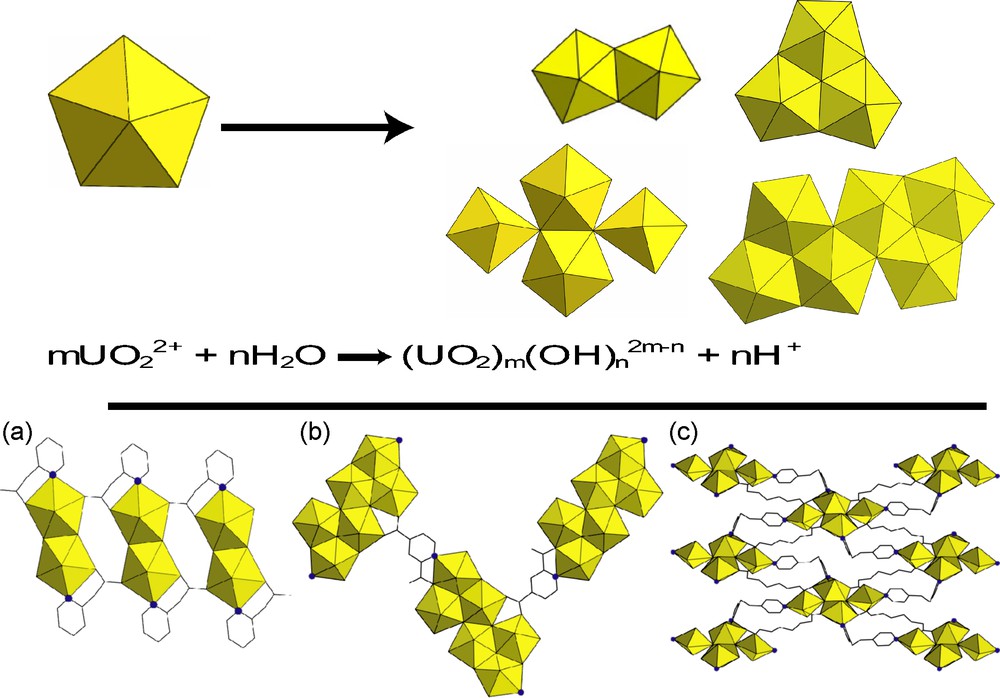
Top: examples of possible secondary building units from uranyl hydrolysis. This speciation is governed by the equation provided; bottom: examples of uranyl containing hybrid materials which highlight the diversity of structures observed; from left, a: {UO2(C7H6NO2)(OH)}n [13]; b: [(UO2)3(μ3-O)(μ3-OH)(μ2-OH)(2,4-pyda)(H2O)2]n·nH2O [40]; c: (UO2)(O)(C8H12O4)(C12H12N2) [12].
Another approach to creating novel uranyl-bearing materials is to reject metal-ligand coordination as the sole means of extending networks and focus on non-covalent interactions between fixed supramolecular entities to achieve new architectures. Herein we describe the assembly of ‘tectons’ – supramolecular entities that contain the necessary molecular fragments to assemble through non-covalent interactions (i.e. ‘synthons’). Synthons may be further defined as “molecular fragments and the associations between them” that in turn approximate the crystal [9]. As an introduction and inspiration for our work, other groups have demonstrated that transition metal perhalo-anions interact predictably with bipryidinium cations (here, both species are tectons) to form a regular synthon () wherein the metal bound halogens form bifurcated hydrogen bonds with the organic cations [16–19]. More recently, we have applied this methodology to uranium chemistry through the substitution of the uranyl cation (UO22+) for divalent transition metals of the [MX4]2− tecton (Fig. 3) [20]. This was first proved feasible through the synthesis of [UO2Cl4](C10H10N2) as will be discussed later.
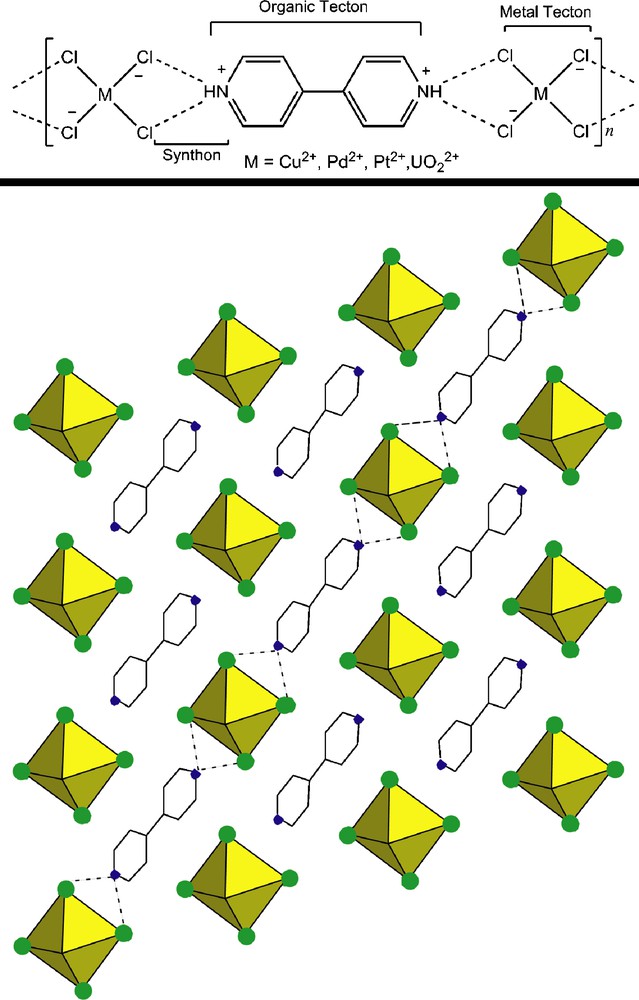
Top: scheme illustrating the bifurcated hydrogen bonding motif; bottom: polyhedral representation of [UO2Cl4](C10H10N2). The protonated nitrogen atoms of the 4,4′-dipyridyl are shown in blue and the chloride ions are shown in green. The dashed lines represent the bifurcated hydrogen bond . Hydrogen atoms have been omitted in this and all subsequent figures.
Beyond supramolecular assembly, this work presents reactions of uranium in acidic, halide-rich media which have additional importance as studies of these systems are particularly relevant to nuclear fuel reprocessing and separations involving hexavalent uranium, as well as geologic disposal of nuclear waste in salt formations [21–23]. Both of these environments may have high halide concentrations and thus exploration of solid-state materials containing the [UO2X4]2− (where X = Cl, Br) entity as a primary building unit are of fundamental interest.
2 Results and discussion
This paper presents an extension of previous work in applying the () synthon to systems containing a uranyl-based tecton ([UO2Cl4]2−) [20]. The first phase we explored, [UO2Cl4](C10H10N2), is an archetype for subsequent phases and is shown in Fig. 3. This hybrid material contains the aforementioned [UO2Cl4]2− tecton and protonated 4,4′-dipyridylamine cation, an organic tecton. These moieties possess the molecular fragments necessary to realize the bifurcated hydrogen bonding (M-X2⋯H-N) synthon. Successive modifications to the organic tecton produced six additional structures, with half of these exhibiting the synthon. This effort, including [UO2Cl4](C10H10N2) and the other compounds discussed, represents a departure from our previous synthetic direction, which focused on metal-ligand coordination in the creation of new hybid materials.
As a rich body of uranyl coordination polymers currently exists [11–14,24–33], and considering the success of this ‘non-covalent’ approach [20], we embarked on further exploration of these supramolecular-based polymeric compounds. In this contribution, we explore a new tecton, namely uranyl tetrabromide ([UO2Br4]2−), a moiety previously observed in the solid state (yet not in this context) [34,35], to demonstrate the utility of supramolecular chemistry when creating families of uranyl materials. The crystal structures of five compounds containing uranyl tetrabromide have been determined. This work includes five examples of organic cations (Scheme 1) and the relevant crystallographic information for these new phases can be seen in Table 1.
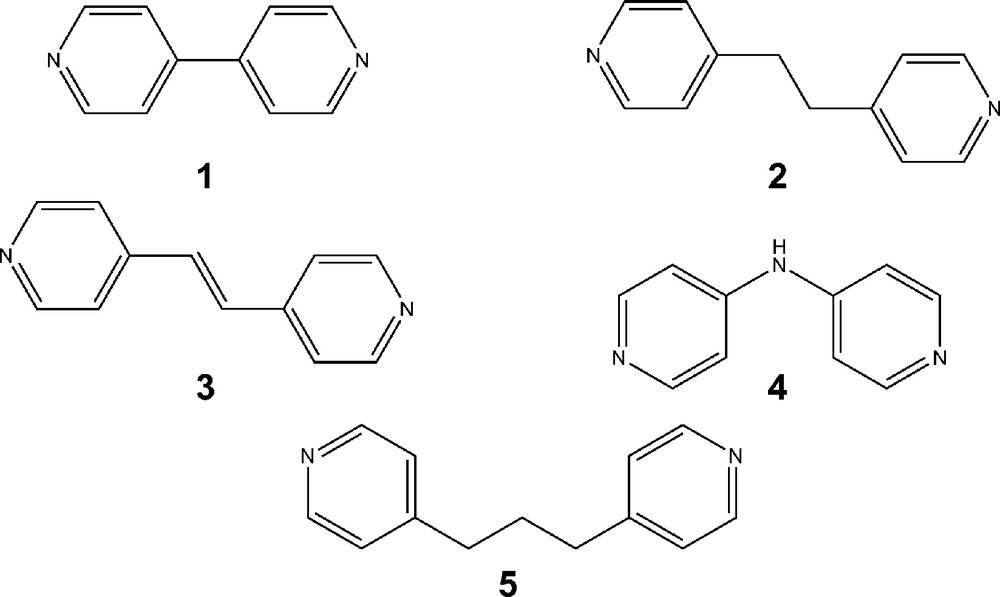
Selected crystal data for compounds 1–5.
1 | 2 | 3 | 4 | 5 | |
Chemical formula | C10H10N2O2UBr4 | C12H14N2O2UBr4 | C12H12N2O2UBr4 | C20H26N6O4UBr6 | C26H32N4O2UBr6 |
Formula weight | 747.87 | 775.88 | 773.87 | 1131.96 | 1150.05 |
Crystal system | Triclinic | Triclinic | Triclinic | Triclinic | Triclinic |
Space group | P-1 | P-1 | P-1 | P-1 | P-1 |
a/Å | 6.8486(6) | 6.9364(17) | 7.0925(6) | 8.1574(4) | 8.6949(10) |
b/Å | 7.3506(7) | 8.236(2) | 8.4033(9) | 9.8222(4) | 9.0059(11) |
c/Å | 9.2054(8) | 9.380(2) | 8.7823(8) | 9.8893(4) | 11.7255(14) |
α° | 85.915(1) | 79.855(4) | 80.634(2) | 94.769(1) | 79.156(2) |
β° | 79.327(1) | 70.449(4) | 73.087(1) | 100.771(1) | 74.365(2) |
γ° | 64.478(1) | 66.877(4) | 73.087(1) | 91.591(1) | 82.360(2) |
V/Å3 | 410.94(6) | 463.8(2) | 453.59(7) | 774.95(6) | 865.12(18) |
Z | 1 | 1 | 1 | 1 | 1 |
Temperature/K | 298(2) | 298(2) | 298(2) | 298(2) | 298(2) |
Dcalc/g cm−3 | 3.022 | 2.778 | 2.833 | 2.426 | 2.207 |
μ (Mo Kα)/mm−1 | 19.595 | 17.368 | 17.758 | 13.006 | 11.647 |
Independent reflections | 2285 | 2565 | 2496 | 4295 | 4628 |
Rint | 0.0268 | 0.0250 | 0.0249 | 0.0361 | 0.0532 |
R1 [I > 2σ(I)] | 0.0255 | 0.0212 | 0.0189 | 0.0331 | 0.0473 |
2.1 Crystal structures 1–5: [UO2Br4]2− phases
Compound 1, [UO2Br4](C10H10N2), crystallizes in the space group P-1 with one crystallographically unique [UO2Br4]2− anion and one protonated 4,4′-dipyridylamine cation, both of which are at centers of inversion. The crystallographically unique U site exists as a central UO22+ with four bromides in the equatorial plane resulting in the square bipyramidal [UO2Br4]2− anion. The uranyl oxygens are at a distance of 1.759 Å from the U center, and the bromide ions are at an average distance of 2.816 Å. This U coordination sphere is typical of that seen in compounds 1–5 presented herein and is thus not discussed in further detail. The pyridinium cation is effectively flat, that is, there is no torsion angle between the planes of the pyridyl groups. The local coordination of 1 is shown in Fig. 4, and we note that it packs in a very similar manner to [UO2Cl4](C10H10N2).[20] There are two interactions in 1 that lead to the bifurcated hydrogen bonding synthon described earlier. These interactions, shown in Table 2, are located between the nitrogen atom of the organic tecton (N1) and the bromine ions of the metal anion complex (Br1 and Br2).
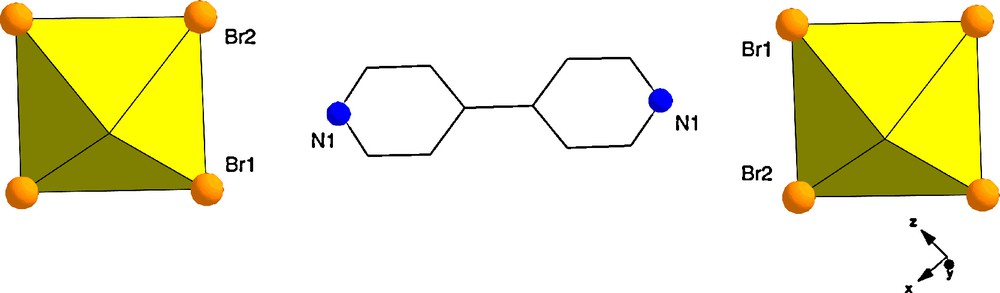
Polyhedral representation of the local structure of 1, atoms which participate in hydrogen bonding are labelled. Bromide ions are shown orange.
Selected hydrogen bonding geometry in compounds 1–5a.
D-H/Å | H⋯A/Å | D⋯A/Å | D-H⋯A/° | ||
1 | N1⋯Br1i | 0.86 | 2.62 | 3.3194(4) | 139.6 |
N1⋯Br2i | 0.86 | 3.08 | 3.704(4) | 131.1 | |
2 | N1⋯Br2ii | 0.86 | 2.77 | 3.456(3) | 137.4 |
N1⋯Br1ii | 0.86 | 2.84 | 3.468(3) | 131.1 | |
3 | N1⋯Br1iii | 0.86 | 2.71 | 3.459(3) | 146.4 |
N1⋯Br2iii | 0.86 | 2.94 | 3.487(3) | 122.9 | |
4 | N1⋯Br1iv | 0.86 | 2.72 | 3.455(4) | 140.0 |
N1⋯Br3iv | 0.86 | 3.06 | 3.620(5) | 124.4 | |
N3⋯OW1v | 0.86 | 1.90 | 2.724(5) | 159.8 | |
N2⋯Br3vi | 0.73(4) | 2.65(4) | 3.374(4) | 171(4) | |
5 | N1⋯Br3vii | 0.86 | 2.41 | 3.221(8) | 157.5 |
N2⋯Br3 | 0.86 | 2.34 | 3.187(6) | 161.1 |
a Symmetry codes: i: x,y + 1,z; ii:-x + 1,-y + 1,-z; iii: x + 1,y,z; iv: -x + 1,-y,-z + 1; v: x,y,z + 1; vi: -x + 1,-y + 1,-z + 1; vii: x,y-1,z + 1.
Compounds 2 and 3, [UO2Br4](C12H14N2) and [UO2Br4](C12H12N2) each belong to the space group P-1 with the uranium of the [UO2Br4]2− and the protonated cations (1,2-bis(4-pyridyl)ethane and trans-1,2-bis(4-pyridyl)ethylene respectively) both located at a center of inversion. The protonated ring of the pyridine is involved in symmetrical bifurcated hydrogen bonding which leads to a 1-D hydrogen bonded network of alternating cations and anions as seen in Figs. 5 and 6. In both, π-π interactions are present with distances of 3.857 and 4.271 Å respectively.
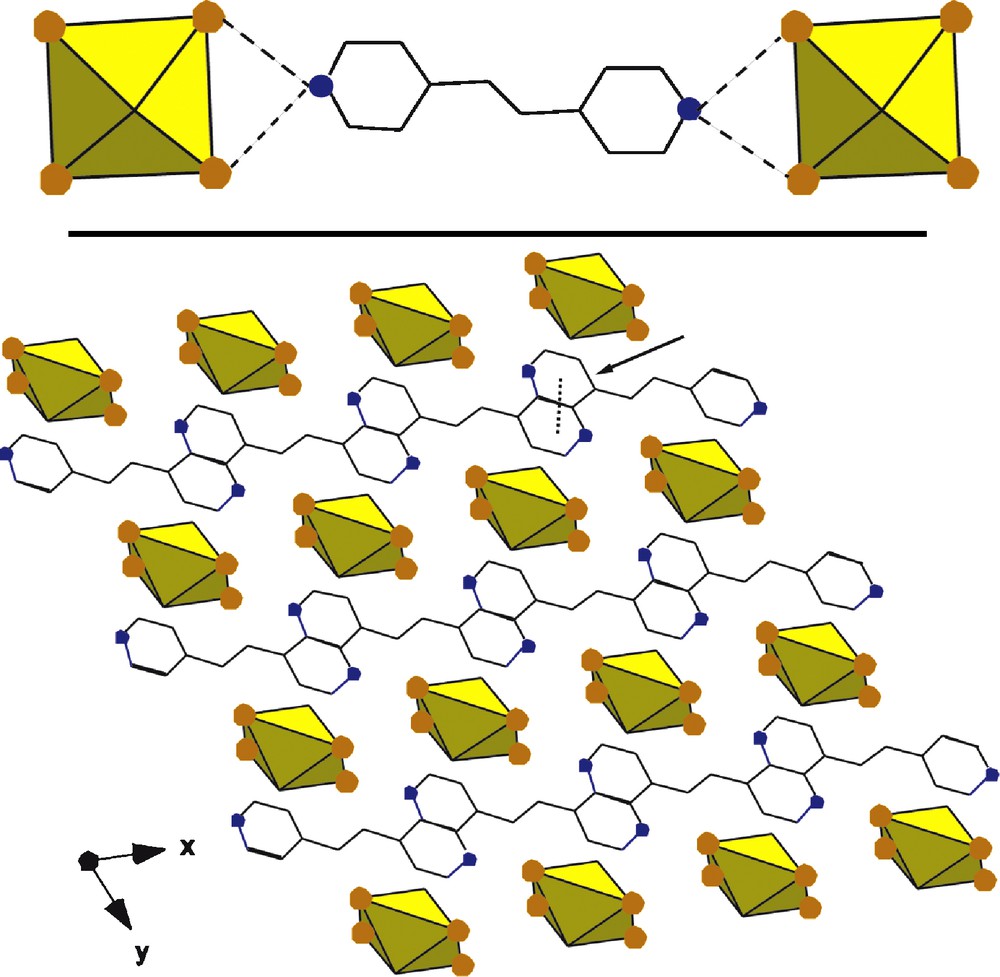
Top: polyhedral representation of 2 showing the local structure. N-Br interactions of 3.468 and 3.456 Å are indicated with dashed lines. Bottom: The packing of one layer of 2 along [0 0 1]. A π-π interaction of 3.857 Å is indicated with an arrow.
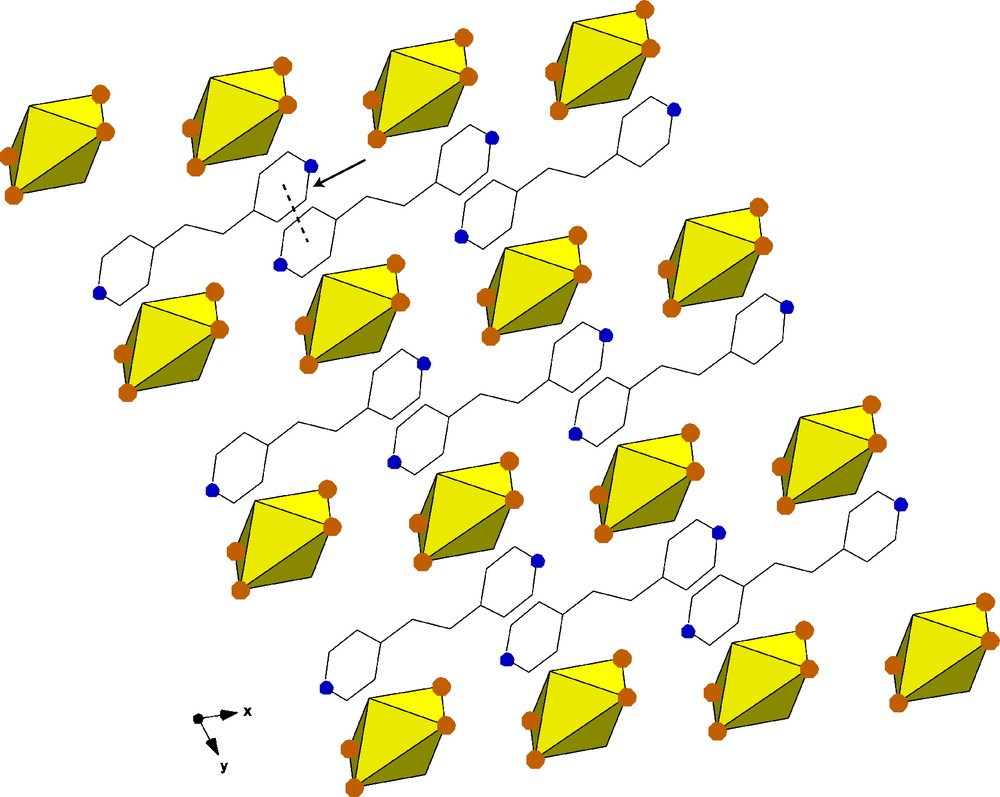
The packing of one layer of 3 along [0 0 1]. A π-π interaction of 4.271 Å is indicated with an arrow.
Compound 4, [UO2Br4](C10H11N3)2·2Br·2H2O crystallizes in the triclinic space group P-1 and differs from the previous three structures in that both free bromide ions and solvent water molecules are present (Fig. 7). As seen in Table 2, there is one significant intermolecular interaction between the protonated 4,4′-dipyridylamine and the [UO2Br4]2−, specifically a hydrogen bond between Br1 and N1. Additional interactions of the organic tecton are exclusively with the free chloride ion (Br3) and the unbound water (OW1).
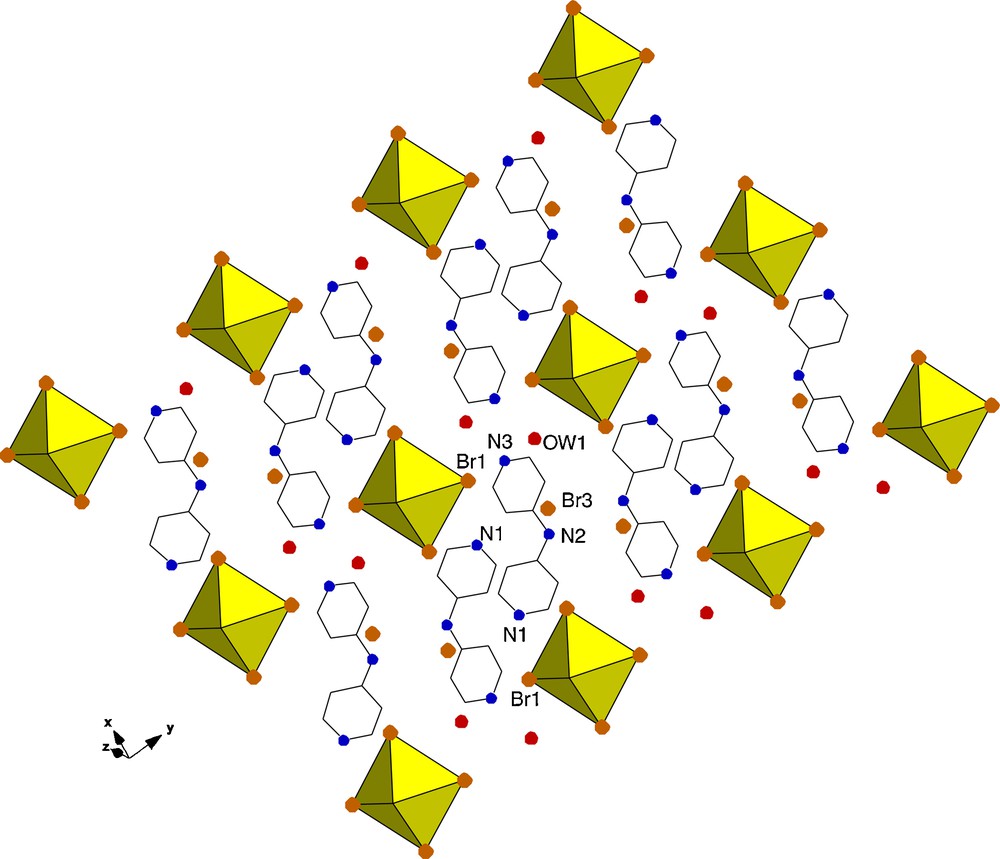
Polyhedral representation of 4 showing the packing, atoms which participate in hydrogen bonding are labelled.
Compound 5, [UO2Br4](C13H16N2)2·2Br, crystallizes triclinic space group P-1 and is shown in Fig. 8. As seen in Table 2, there is no significant intermolecular interaction between the protonated 4,4′-trimethylene dipyridine and the [UO2Br4]2−, rather, the organic cation interacts exclusively with the free chloride ion (Br3). Further, there is a π-π interaction of 3.677 Å between adjacent pyridyl rings.
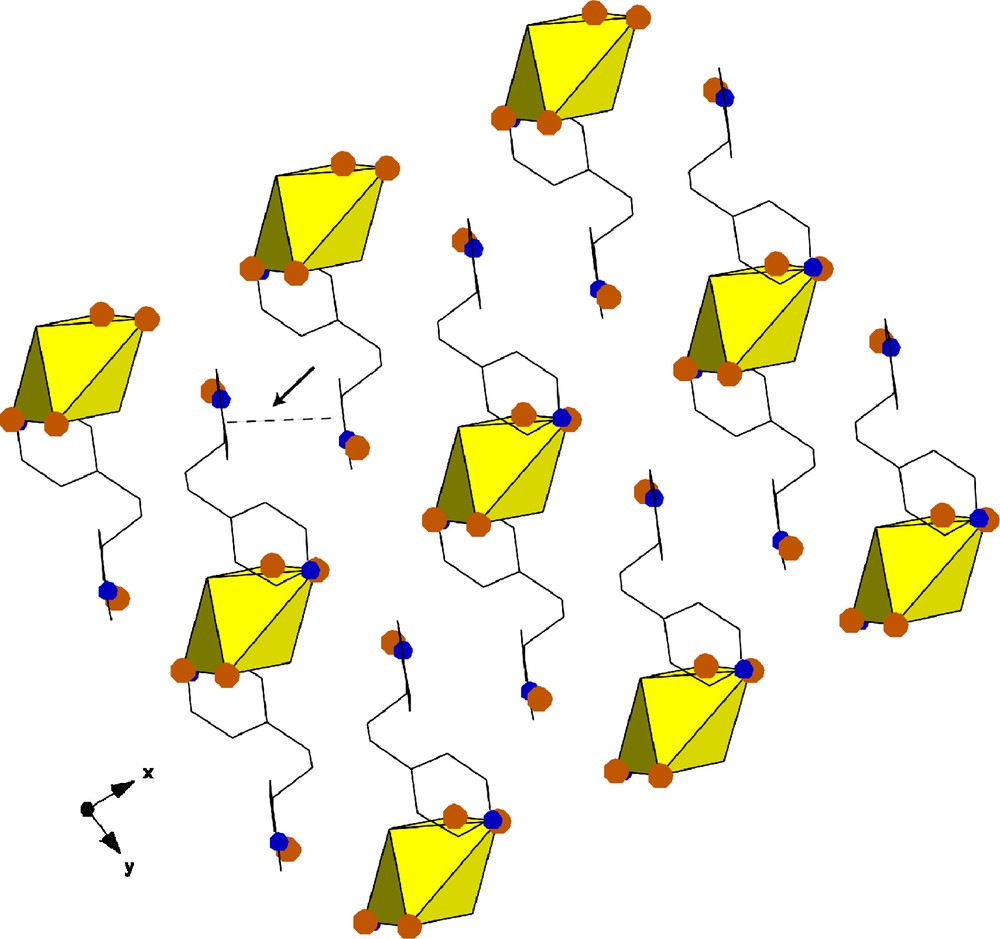
Polyhedral representation of 5 showing the packing along [0 0 1]. A π-π interaction of 3.677 Å is indicated with an arrow.
2.2 Discussion of compounds 1–5
Whereas the above compounds all contain the [UO2Br4]2− tecton, only structures 1–3 exhibit the U-Br2⋯H-N synthon. This is expected and follows our previous studies [20] as 1–3 represent the only structures discussed above where the organic tectons are linear. In all three, a distance (here the ) is on the same order as the sum of the van der Waal radii (3.40 Å [36]) for the respective atoms, however only 1 has an interaction within this distance. It is additionally clear that the bifurcated hydrogen bonding is more symmetric in 2 and 3 then in 1 and their [UO2Cl4]2− containing analogues.
In structures 4 and 5, the non-linear organic tectons, while possessing the correct pyridyl functionality to perpetuate the synthon, conceivably lack the ability to pack in a manner that preserves the bifurcated hydrogen-bonding pattern. As such, other interactions prevail in organizing the tectons. It is noteworthy that 5 packs remarkably different than its previously reported [UO2Cl4]2− analogue [20], and also incorporates free bromide ions.
2.3 Fluorescence
Fluorescence spectra were collected for materials 1–5 (with all of the samples explored via excitation of the uranyl centers at 365 nm). Interestingly, there is a distinct lack of uranyl emission from the [UO2Br4]2− phases reported herein. Fluorescence experiments were conducted on a Shimadzu RF-5301 PC spectrofluorophotometer using a xenon lamp (emission wavelengths 400–650 nm; excitation slit width 1.5 nm; emission slit width 1.5 nm; high sensitivity). Efforts to determine a mechanism for this phenomenon are underway yet one could speculate at present that charge transfer interactions between the uranyl cation and the softer bromide ions (versus chloride ions) may have some influence.
3 Conclusion
We have demonstrated the use of the uranyl tetrabromide anion as a tecton that can be used towards the crystal engineering of hexavalent uranium bearing materials. The bifurcated hydrogen bonding synthon described above forms a somewhat predictable supramolecular architecture when a small subset of linear, organic tectons are considered. Though matching various organic tectons with uranyl tetrahalide ([UO2X4]2−) tectons has proven useful in creating specific structure types, a more narrow range of diversity is present in these phases as compared to materials made through metal-ligand coordination.
As the many forces which contribute to the organization of molecules and ions in the solid state are not always apparent when attempting syntheses of new materials, known supramolecular synthons are a convenient way of summarizing major interactions. As these synthons are explored in the synthesis of novel uranium-organic hybrid materials, we hope to move away from chance results and towards creating systems of predictable structure types. Though we are clearly able to predict the some of the main supramolecular interactions observed within this study, we must further consider if this work constitutes ‘true’ crystal engineering, a term pervasive in the literature. Regardless, we feel that this system is ripe for further exploration, as it will yield more information about the self-assembly [UO2X4]2− tectons with protonated organic amines and potentially, a predictable and tunable uranium-organic hybrid system.
4 Experimental
4.1 Synthesis
Caution: whereas the uranium oxyacetate dihydrate (UO2(CH3COO)2·2H2O) used in this study consists of depleted U, standard precautions for handling radioactive and toxic substances should be followed. The uranium was purchased from Alpha Aesar and was used without further purification. All organics were purchased from Sigma Aldrich and used without any further purification except for the 4,4′-dipyridylamine which was synthesized according to a published procedure [37]. The compounds 1–5 crystallized at room temperature from a mixture of uranium oxyacetate, organic amine (as seen in Scheme 1), water and HBr.
4.1.1 [UO2Br4](C10H10N2) (1)
Compound 1 was prepared by dissolving 0.255 g of UO2(CH3COO)2·2H2O in water (2.5 mL) and HBr (0.75 mL, 48% in H2O) in a 25 mL Erlenmeyer flask. To this yellow solution, a solution of 4,4′-dipyridyl (0.211 g) in water (2.5 mL) and HBr (0.75 mL, 48% in H2O) was added. The resulting mixture was evaporated using gentle heat to an approximate volume of 4 mL and allowed to cool. The flask was then covered with a piece of parafilm into which several holes were punched. After one month, yellow, X-ray quality crystals were obtained.
4.1.2 Compounds 2–5
The remaining four compounds were prepared under the same conditions. At various intervals, X-ray quality crystals were obtained. Specific synthetic details are included in the ESI, however, it is noted here that 3 and 5 were not obtained in the same yield and purity as were the remaining compounds. The crystallization product that contained 3 was a mixture of white solids and small yellow crystals of 3. The synthesis of 5 was unique in that no large crystals (crystal dimensions greater than 1 mm) were observed, as were typical in these syntheses. Rather, the crystal used to obtain a data set for 5 was taken from a crystalline reaction product that contained much smaller crystals. As such, we obtained powder X-ray diffraction data for this product to confirm phase purity and this can be seen in the ESI.
4.2 X-Ray crystallography
Single crystals of 1–5 suitable for diffraction were obtained by cleaving small pieces of the large (dimensions greater than 1 mm) crystals formed during the crystallization of these materials, excepting 3 and 5, which were of suitable size for diffraction without further processing. The crystal structures were determined via single crystal X-ray diffraction and the crystallographic data are summarized in Table 1. Intensity data were collected on a Bruker SMART diffractometer equipped with an APEX II CCD Detector. Data processing was performed using Bruker Software including absorption corrections with SADABS [38]. The structures were solved using direct methods and refinements were carried out using SHELXL-97 [38] within the WINGX [39] software suite. All non-hydrogen atoms were refined anisotropically and all hydrogen atoms were introduced in idealized positions and refined isotropically with a riding model, with the exception of the hydrogen atoms on the unbound solvent water and H2 (bridging amine) atom in 4. The H2 atom in 4 was found in the Fourier-difference map and refined isotropically, while the hydrogen atoms on the unbound water were omitted from the final model. The thermal parameters for C1 and C2 in 1 are slightly elongated (see ESI), yet in light of satisfactory refinement and structural metrics, we have opted not to model what is likely minor disorder. The crystallographic data in CIF format have been uploaded to the CCDC and have been assigned reference numbers 757655 – 757659.
Acknowledgements
This work was supported by the National Science Foundation (DMR-0348982 and DMR-0419754), and the Chemical Sciences, Geosciences and Biosciences Division, Office of Basic Energy Sciences, Office of Science, Heavy Elements Program, U.S. Department of Energy, under grant DE-FG02-05ER15736 at GWU. Additional support was provided as part of the Materials Science of Actinides, an Energy Frontier Research Center funded by the U.S. Department of Energy, Office of Science, Office of Basic Energy Sciences under Award Number DE-SC0001089.
Appendix A Supplementary data
ORTEP illustration of 1. Ellipsoids are shown at 50% probability level. Superscript denotes symmetry transformations i = -x,-y,-z and ii = -x+2,-y+1,-z+1. ORTEP illustration of 2. Ellipsoids are shown at 50% probability level. Superscript denotes symmetry transformations i = x,-y,-z and ii = -x,-y+1,-z+1. ORTEP illustration of 3. Ellipsoids are shown at 50% probability level. Superscript denotes symmetry transformations i = -x,-y,-z and ii = -x-1,-y+1,-z+1. ORTEP illustration of 4. Ellipsoids are shown at 50% probability level. Superscript denotes symmetry transformation i = -x+2,-y,-z+2. ORTEP illustration of 5. Ellipsoids are shown at 50% probability level. Superscript denotes symmetry transformations i = -x,-y,-z. PXRD of 5 with calculated pattern overlaid in blue. We acknowledge a small impurity.