1 Introduction
Coordination complexes may exhibit chirality owing to an asymmetric arrangement of ligands around the metal [1]. It is well known for example that certain tris(chelate) [M(AA)3]n+ complexes may be separated into Δ and Λ enantiomers. When chiral nonracemic ligands are used, one isomer may be formed preferentially [2,3]. This occurrence has been termed predetermination of chirality-at-metal [2,4]. Chiral-at-metal complexes normally find application in the enantioselective catalysis; indeed a well-defined dissymmetry should be strongly communicated to prochiral co-ligands [5]. As matter of fact, a metal complex showing significant catalytic activity must contain free or potentially free coordination sites where substrates and reagent species may interact in close proximity; then, for matching such a criterion, a good design of a suitable chiral ligand which works as a precursor for diastereomerically pure chiral-at-metal complexes plays an essential role.
For linear tetradentate ligands in an octahedral coordination environment, three geometrical isomers may be formed (trans, cis-α and cis-β, Fig. 1).

(Color online.) Octahedral-based geometric isomers for a linear tetradentate ligand.
The trans structure has C2v symmetry and therefore not chiral-at-metal. The C1-symmetric cis-β and C2-symmetric cis-α structures have enantiomers with Δ and Λ helicity, and most importantly for the purposes of enantioselective catalysis, cis orientated co-ligands, (X, Fig. 1). If the X ligands mutually differ, either as two monodentate ligands or in a bidentate ligand, then further isomers are possible [6].
In the aim of preparation of rigid chiral precursor in chiral Schiff base ligands, most of researchers used either pure or racemic molecular backbones [7–18]. For example, the use of a chiral biaryl diamine structure in tetradentate ligands was explored by Lions and Martin in 1957 [7] and 1960 [8]. They found that these ligands were capable to coordinate a given metal in a non-planar fashion without any significant ligand strain, and synthesized an octahedral ruthenium(II) complex to which they assigned cis-α geometry. However, they did not pay proper attention to the role played by the achiral backbone groups in the synthesis of rigid chiral precursors.
Herein, we report the crystal and molecular structure of the precursory 1,2-bis(2′-nitrophenoxy)-4-methylbenzene prepared from achiral molecule (4-methylcatechol), in which the two phenoxy groups are placed to one another in such a way that the molecule is C1 symmetrical, i.e. chiral (Scheme 1). The chiral conformation of 1,2-bis(2′-nitrophenoxy)-4-methylbenzene after reduction is maintained in the octahedral zinc(II) and cobalt(II) complexes prepared from the Schiff base. This implies that in the Schiff base ligand, the two α-diimine systems shape an octahedral cavity having the same chirality of the precursory diamine.
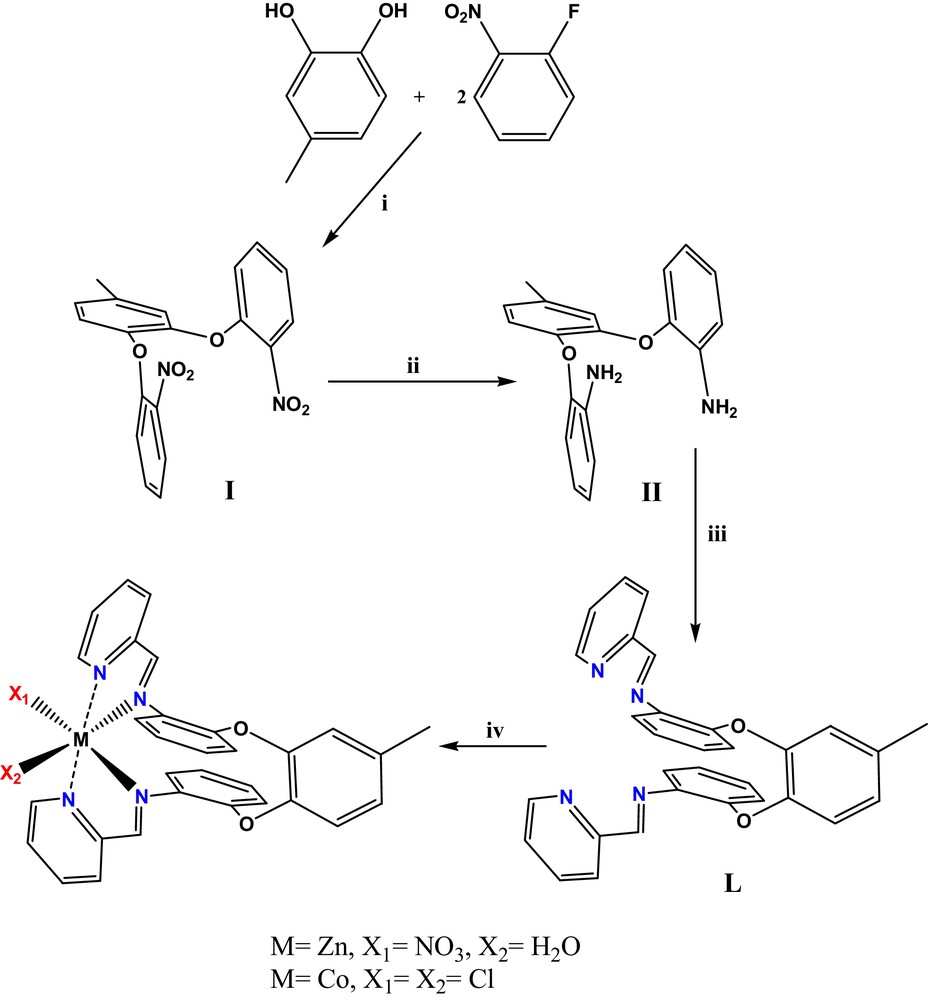
(Color online.) Synthetic route used for the preparation of compounds: i: K2CO3/DMF, Xylen; ii: dust Zn/NH4Cl/EtOH, H2O; iii: 2-Pyridinecarboxaldehyde/EtOH; iv: for Zinc (II) complex: Zn(NO3)2.6H2O/EtOH; for Cobalt (II) complex: CoCl2.6H2O/EtOH.
2 Results and discussion
1,2-bis(2′-nitrophenoxy)-4-methylbenzene (I) was prepared by an SNAr reaction between 1-fluoro-2-nitrobenzene and 4-methylcatechol. The IR spectrum reveals absorption bands at ca 1521 and 1347 cm−1 due to symmetric and asymmetric stretching of the NO2 group [19,20]. In the 1H NMR spectrum, the absorption signals of aromatic protons appear in the region of 6.9–7.9 and the methyl group appears at 2.38 ppm. The 13C NMR in CDCl3 showed the expected 16 aromatic signals.
Aromatic nitro compounds can be reduced in high yield to the corresponding diamines using zinc metal and NH4Cl in H2O/EtOH [21]. After reduction, the characteristic absorptions of nitro groups disappeared and the amino groups showed NH stretching bands at 3468 and 3376 cm−1. In the 1H NMR spectrum, all the aromatic protons of 1,2-bis(2′-aminophenoxy)-4-methylbenzene (II) resonated in the 6.6–7.3 ppm region. Hydrogens of the methyl group appeared at 2.29 ppm and the signal appearing at 3.69 ppm corresponds to the amine group. Comparing the 13C NMR spectrum of 1,2-bis(2′-aminophenoxy)-4-methylbenzene (II) with the spectrum of the precursors 1,2-bis(2′-nitrophenoxy)-4-methylbenzene (I), the 13C absorptions of the central three benzene rings move downfield as a result of the change to the electron-donating amino groups from the electron-withdrawing nitro groups [19,20].
The reaction of reduced form of 1,2-bis(2′-nitrophenoxy)-4-methylbenzene (I) with 2-pyridinecarboxaldehyde leads to the novel unsymmetric Schiff base ligand [L]. This ligand possesses a chiral octahedral pocket, suitable to host a proper metal ion. Then, the reaction of the ligand [L] with zinc(II) nitrate and cobalt(II) chloride yields the octahedral chiral Zn and Co complexes (Scheme 1).
The chemical structure of the ligand was confirmed by elemental analysis and IR, 1H and 13C NMR spectroscopy, with results in good agreement with the designed compounds. The formation of Schiff base ligand is evidenced by the presence of a strong IR band at ∼ 1609 cm−1 due to ν(CN), while no bands attributable to ν(CO) or to ν(NH2) have been detected. The bands at 1584 and 1488 cm−1 of the pyridine ring vibrations are also present [22]. The 1H NMR spectrum are consistent with the IR spectroscopy. The 1H NMR spectra in CDCl3 show a peak at ∼ 8.4 ppm for ligand corresponding to the imine protons.
The prepared complexes are stable in air. The presence of ν(CN) bands in the correct positions for Schiff base linkages of this kind, and the absence of CO and NH2 indicate that the required macroacyclic Schiff base complexes have indeed formed. The IR spectra exhibit medium to strong bands at ∼ 1598 and ∼ 1485 cm−1 as expected for the two highest energy pyridine ring vibrations. The shift of the imine and pyridine bands by complexation suggests coordination via the imine and pyridine nitrogen atoms. The diamagnetic zinc complex was studied by 1H NMR and 13C NMR. The 1H and 13C NMR were run immediately after solution in DMSO-d6 and gave the expected simple spectrum, indicating the integrity of the complex. The spectra obtained after 12, 24 and 120 h were similar to the initial spectra indicating that the complex is stable in solution. The 1H NMR spectrum of complex shows a peak at ∼ 9.2 ppm due to the formation of the iminic bond. The 13C NMR spectrum show 18 signals for [Zn(L)(NO3)(H2O)](NO3) complex. The peak at 163.2 ppm, assignable to the imine carbon atoms, confirms the presence of the Schiff base in the complex.
The 1H NMR paramagnetic spectra of CoLCl2, was measured in the range from +120 to −120 ppm. The spectrum of the complex is different with those of free ligand due to their paramagnetism, which complicated spectrum interpretation. It has been reported that in the 1H NMR spectrum of Co(salen) complexes, the most upfield shifted resonances which are usually weak and broad, can be assigned to the hydrogen in CHN group [23]. In the 1H NMR spectrum of present Co(II) complex the latter signal was observed at −10.98 ppm and −10.91 ppm. Two single 1H imine resonances for CoLCl2 are due to the non-quivalance of the two-imine environments.
Single crystals suitable for X-ray analysis 1,2-bis(2′-nitrophenoxy)-4-methylbenzene (I) Was obtained from CH3CN. The X-ray crystal structure analysis of the selected single crystal of the 1,2-bis(2′-nitrophenoxy)-4-methylbenzene(I) (Fig. 2) shows that the two phenyl groups linked to 4-methylcatechol are opposite to one another so giving rise to a rigid, chiral molecule. Then, this molecule can exist in two enantiomeric forms. The molecule does not show any crystallographic symmetry in the solid state. Steric repulsion between the ortho hydrogen atoms (between H5 and H8 and between H11 and H14) and also between two NO2 groups is a probable cause of a high rotational barrier in solution (Scheme 2). Such a high-energy barrier is a probable consequence of the size of oxygen atoms. In order to give support to this claim, theoretical calculations (ΔH) at the DFT-B3LYP/cc- level carried out for 1,2-bis(2′-nitrophenoxy)-4-methylbenzene (I) and tpS2(NO2)2 that had been synthesized by Sellmann et al. [24]. The difference of two molecules is existence of sulfur atom in tpS2(NO2)2 and oxygen atom in 1,2-bis(2′-nitrophenoxy)-4-methylbenzene (I). The calculations showed that rotational barrier for tpS2(NO2)2 need low energy, probably because both size of sulfur atom and SC bond length (∼ 1.78 Å), produce a low steric repulsion between the ortho hydrogen atoms as well as between NO2 groups. Remember that the flat structure is the best status for these compounds because in this status p orbitals of sulfur atoms or oxygen atoms can overlap with phenyl ring. The optimized structure of 1,2-bis(2′-nitrophenoxy)-4-methylbenzene (I) [1,2-bis(2′-aminophenoxy)-4-methylbenzene (II)] are similar (Fig. 3). Obviously, in 1,2-bis(2′-aminophenoxy)-4-methylbenzene (II) the steric constrains which causes the existence of a high-energy rotational barrier are due also in this molecule to the steric repulsion between the ortho hydrogen atoms and between two NH2 groups (Scheme 3).
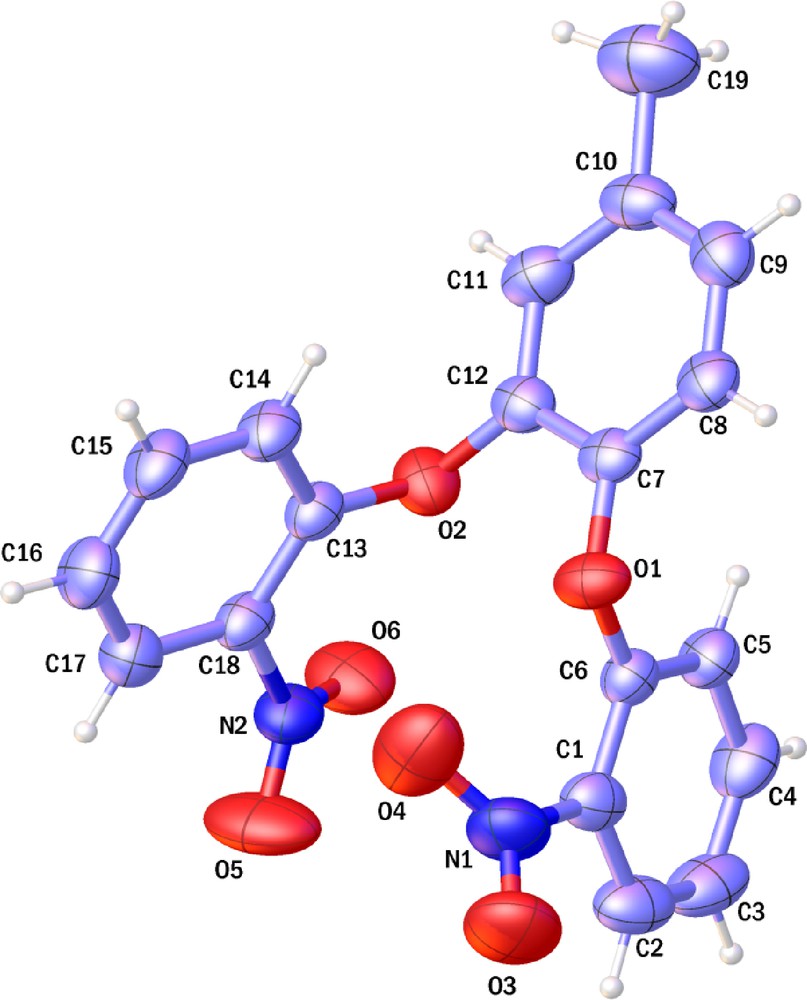
(Color online.) ORTEP representation of 1,2-bis(2′-nitrophenoxy)-4-methylbenzene (I). Displacement ellipsoids are drawn are the 50% probability level and H atoms are shown as small spheres of arbitrary radii.
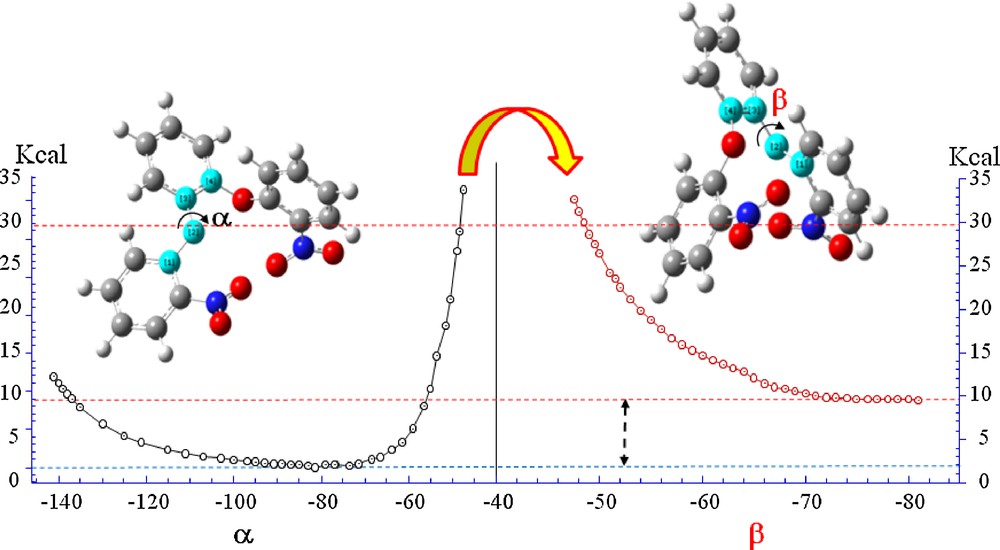
(Color online.) Rotational energy curves computed at B3LYP/6-31 + G(d,p) level for 1,2-bis(2′-nitrophenoxy)benzene (I) around α and β dihedral angles. 4-methyl group has not been taken into consideration.
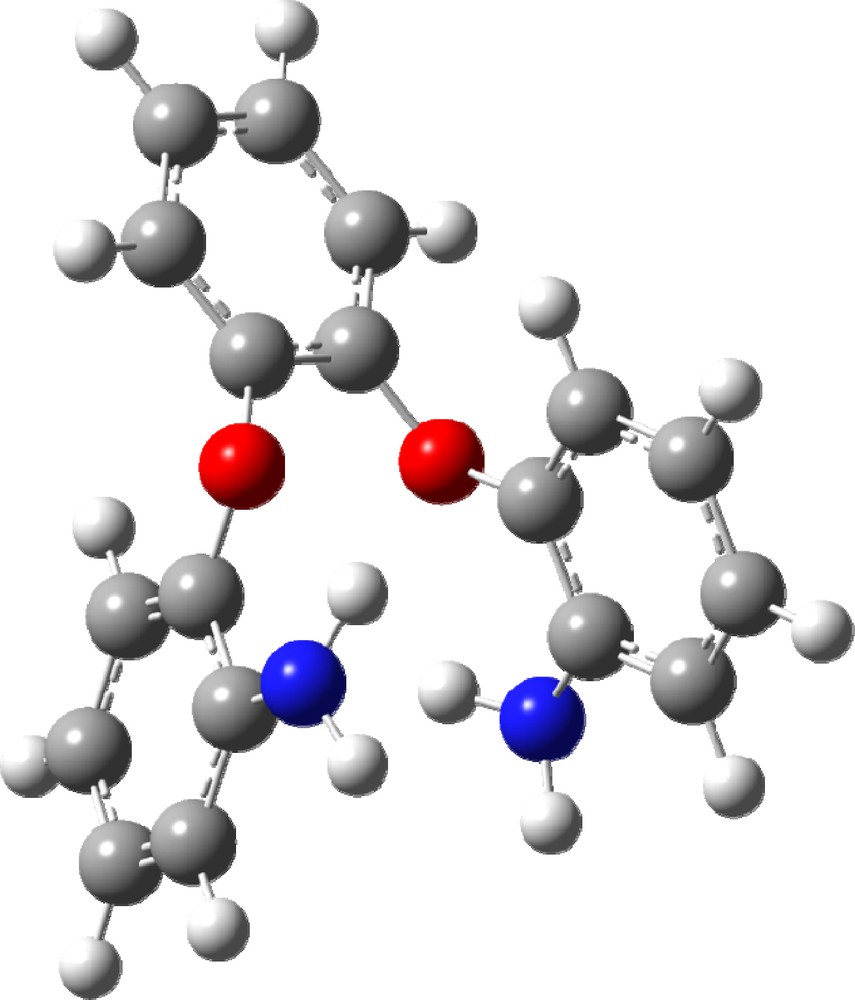
(Color online.) Optimized molecular structure of 1,2-bis(2′-aminophenoxy)-4-methylbenzene (II).
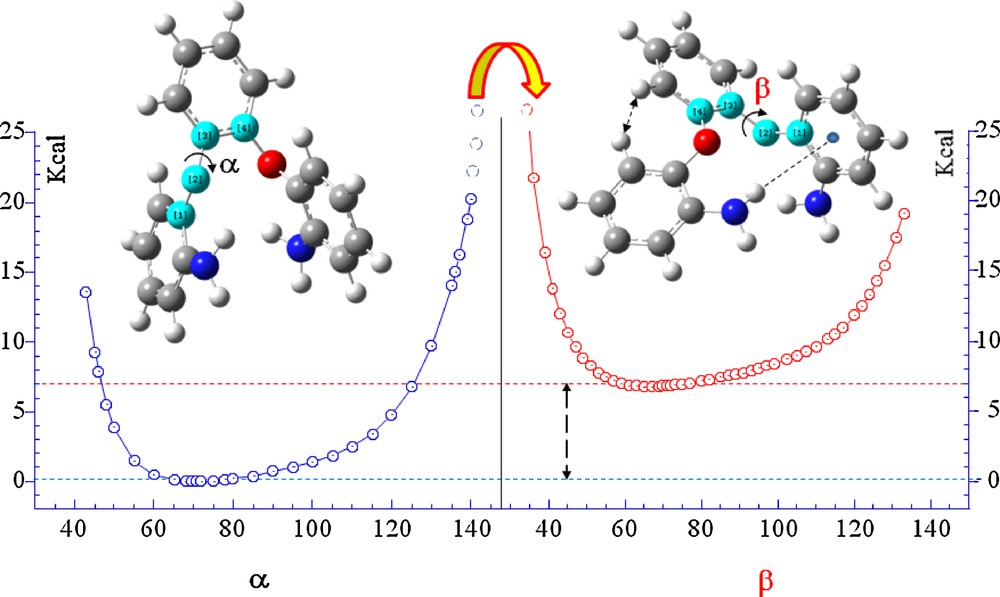
(Color online.) Rotational energy curves computed at B3LYP/6-31 + G(d,p) level for 1,2-bis(2’-aminophenoxy) benzene (II) around α and β dihedral angles without the -4-methyl group.
Molecular structures of the complexes [ZnL(NO3)(H2O)]NO3 and CoLCl2 also have been established by X-ray crystallography. The molecular structure of [ZnL(NO3)(H2O)]NO3 and CoLCl2 are represented on Figs. 4 and 5. The geometry around the metal atom in [ZnL(NO3)(H2O)]NO3 and CoLCl2 are octahedral with the Schiff base ligand bound to the metal centre in a cis-α configuration. Two remaining coordination sites are occupied as cis by two O-bound H2O and NO3 molecules in zinc complex and by two Cl atoms in cobalt complex; as said above, this is important structural aspect in many metal catalysts. The dihedral angles between the phenoxy groups and 4-methylcatechol in [ZnL(NO3)(H2O)]NO3 complex are 82.41 and 85.03 and in CoLCl2 complex are 85.46, 97.58 and 81.37. For both complexes both Δ and Λ enantiomers were observed in solid state (Scheme 4). As we know, cobalt complexes have been widely used in catalysis. Therefore, a cyclic voltammetric electrochemical study of cobalt complex was performed in the potential range of −1.5 to 1 volts. A representative cyclic voltammogram is shown on Fig. 6 [Epc (V): −0.90, Epa (V): −0.85, ΔEp (V): 0.05, E1/2 (V): −0.875]. The cobalt complex shows reversible electrode responses which is due to the cobalt(III)/cobalt(II) couple [32].
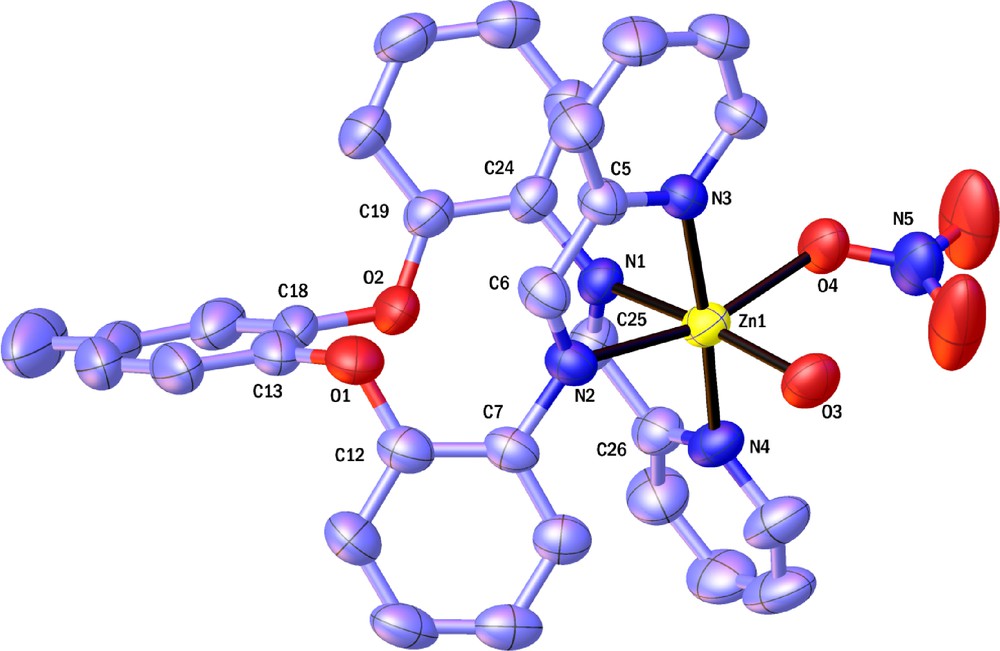
(Color online.) ORTEP representation of Λ-cis-α- [ZnL(NO3)(H2O)]NO3. Displacement ellipsoids are drawn are the 50% probability level. The H atoms and counter ion (NO3) are omitted for clarity.
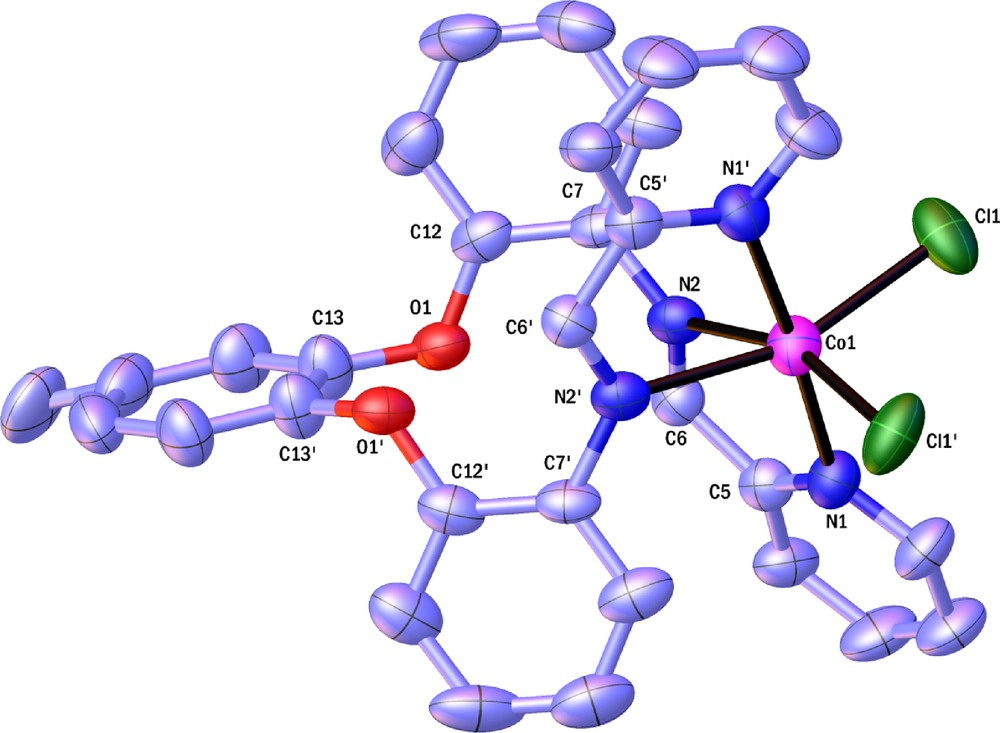
(Color online.) ORTEP representation of Λ-cis-α- CoLCl2. Displacement ellipsoids are drawn are the 50% probability level. The H atoms are omitted for clarity.
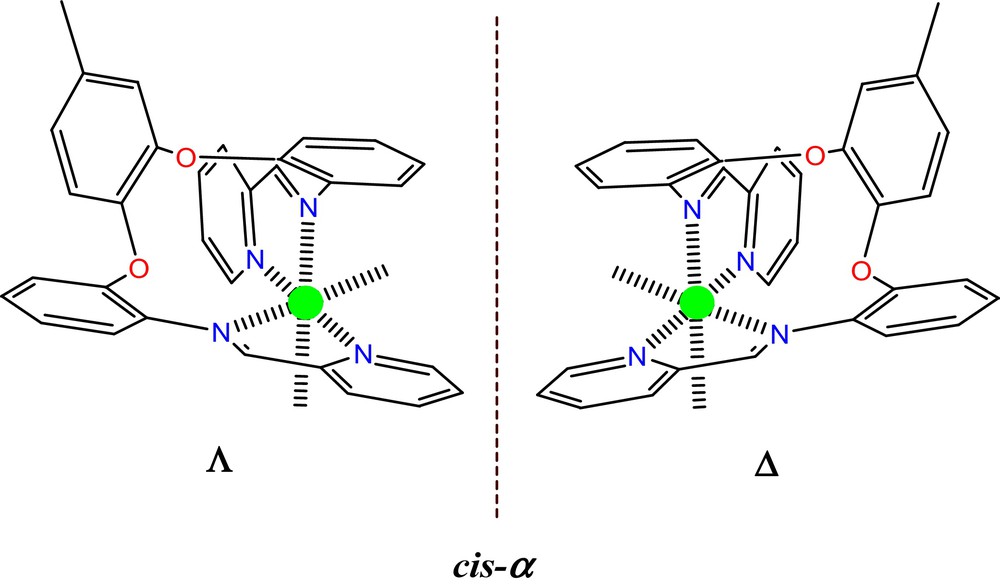
(Color online.) View of the enantiomeric pair of complexes (Λ-enantiomer, left) and 2 (Δ-enantiomer, right) with mirror symmetry.
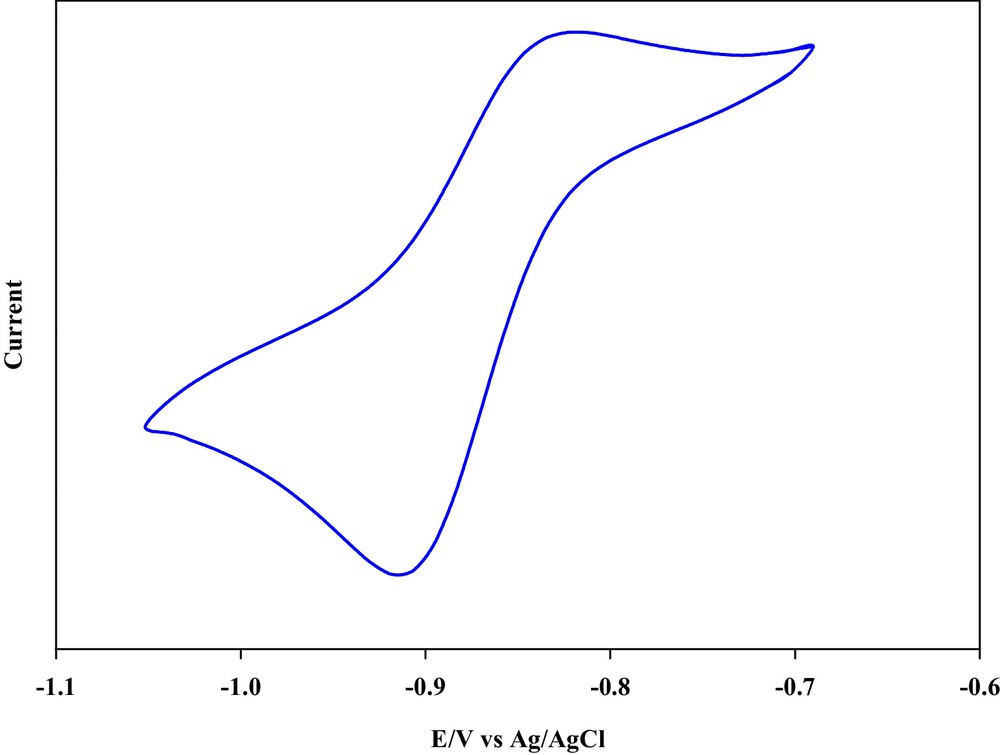
Cyclic voltammogram of Co complex in DMSO at 298 K and 50 mVs−1 scan rate.
In conclusion, both 1,2-bis(2′-nitrophenoxy)-4-methylbenzene (I) and 1,2-bis(2′-aminophenoxy)-4-methylbenzene (II) are rigid chiral molecules in which the rotational barriers are high enough to allow the existence of each enantiomer as an esoterically pure compound. This means that the introduction of a chiral auxiliary in the molecular skeleton (i.e. an enantiomerically pure alkyl substituent instead of -4-methyl group) changes the enantiomers in a couple of separable diastereomers. Each of these diastereomer could provide the corresponding Schiff base, which could work as a chiragen ligand [25]. Then the future work will be oriented in this direction, having in mind the importance of zinc complexes in biological systems as well as of cobalt complexes in catalysis.
3 Experimental
3.1 Materials and physical measurements
1H NMR and 13CNMR spectra were recorded at 298 K on a Bruker ARX-300 spectrometer. Chemical shifts (δ, ppm) were referred to SiMe4. IR spectra were obtained as Nujol mulls on KBr plates by using a Perkin–Elmer FTIR 1720 spectrometer. Solvents were of reagent grade and were purified by the usual methods. 4-Methylcatechol, 1-fuoro-2-nitrobenzene, and 2-pyridinecarboxaldehyde were obtained from Merck Chem. Co. and used without further purification.
3.2 Cyclic voltammetry
The CV measurement was carried out on autolab electrochemical analyzer model PGSTAT30 (Eco Che-mie, Utrecht, The Netherlands) controlled by a microcomputer with GPES 4.9 software in DMSO solutions at room temperature (25 °C) under nitrogen atmosphere using 0.1 M tetrabutylammonium tetrafluoroborate as supporting electrolyte. A glassy carbon working electrode, a platinum auxiliary electrode and an Ag/AgCl reference electrode was used to obtain cyclic voltammogram.
3.3 X-ray crystallography
X-ray diffraction data were collected on a Bruker Smart Apex II CCD diffractometer with graphite monochromatic Mo Kα radiation (λ = 0.71073 Å) at room temperature. Data collection, cell refinement, data reduction and absorption correction were performed by multi-scan methods by means of the Bruker software [26]. The structures were solved by direct methods using SIR2004 [27]. The non-hydrogen atoms were refined anisotropically by the full-matrix least-squares method on F2 using SHELXL [28]. All the H atoms were introduced in calculated positions and constrained to ride on their parent atoms.
3.4 Synthesis of 1,2-bis(2′-nitrophenoxy)-4-methylbenzene (I)
4-methylcatechol (12.4 g, 0.1 mol) was dissolved in DMF (100 mL)/xylene (20 mL) before K2CO3 (42 g, 0.3 mol) and 1-fluoro-2-nitrobenzene (28.2 g, 0.2 mol) were added. The mixture, refluxed at 130–135 °C under a dinitrogen atmosphere for 16 h with stirring, was then allowed to cool and poured into H2O (500 mL), which afforded an oil residue. The oil was isolated. Pure product was obtained as yellow crystals by recrystallization from ethanol. Yield: 34.4 g (94%). Anal. Calcd for C19H14N2O6: C, 62. 30; H, 3.85; N, 7.65. Found: C, 62.19; H, 3.83; N, 7.70%. IR: 1521, 1347 (NO2), 1225 (COC str). 1HNMR (CDCl3, 300 MHz) δ 6.915–7.876 (m, 11H, aromatics), 2.380 (s, 3H, CH3) ppm. 13CNMR (CDCl3, 300 MHz) δ 20.937 (CH3), 118.322, 118.763, 122.380, 122.738, 122.812, 122.989, 125.624, 125.638, 126.998, 134.248, 134.292, 137.013, 143.247, 145.542, 150.382, 150.710 (aromatic rings) ppm.
3.5 Synthesis of 1,2-bis(2′-aminophenoxy)-4-methylbenzene (II)
A mixture of 1,2-bis(2′-nitrophenoxy)-4-methylbenzene (3.66 g, 10 mmol), NH4Cl (1.07 g, 20 mmol) in EtOH (100 mL) was heated to boiling and zinc dust (2 g, 30 mmol) was added in 0.1 g portions at intervals of several minutes. The mixture was reflux for 24 h. The solution was evaporated to dryness and the residue extracted with H2O/CHCl3. The organic layer was evaporated to yield organic oil. Yield: 2.79 g (91%). Anal. Calcd for C19H18N2O2: C, 74.49; H, 5.92; N, 9.14. Found: C, 74.22; H, 6.06; N, 9.14%. IR: 3468, 3376 (NH2), 3034 (CH)ar. 1HNMR (CDCl3, 300 MHz) δ 6.628–7.260 (m, 11H, aromatics), 3.693 (b, 4H, NH2), 2.292 (s, 3H, CH3) ppm. 13CNMR (CDCl3, 300 MHz) δ 20.669 (CH3), 116.126, 116.205, 116.983, 117.983, 118.094, 118.141, 119.907, 120.014, 123.573, 123.989, 124.269, 134.141, 137.478, 137.805, 143.642, 144.200, 144.414,146.777 (aromatic rings) ppm.
3.6 Synthesis of Schiff base ligand (L)
2-Pyridinecarboxaldehyde (0.22 g, 2 mmol) in dry EtOH (25 mL) was slowly added to a stirred solution of 1,2-bis(2′-aminophenoxy)-4-methylbenzene (II) (0.31 g, 1 mmol) in dry EtOH (25 mL). The yellow solution was stirred for 4 h. The solvent volume was reduced, cooled in an ice bath for 5 h and the yellow precipitate formed was filtered off and dried in vacuo. L: Yield: 0.25 g (52%). Anal. Calcd for C31H24N4O2: C, 76.84; H, 4.99; N, 11.56. Found: C, 76.69; H, 5.09; N, 11.43%. IR: 1609 (CHN)imi, 1584 (CHN)py. 1HNMR (CDCl3, 300 MHz) δ 8.438 (s, 2H, CHN), 6.023–7.724 (m, 19H, aromatics), 2.241 (s, 3H, CH3) ppm. 13CNMR (CDCl3, 300 MHz) δ 20.438 (CH3), 151.27–114.83 (aromatic and pyridine rings) ppm.
3.7 Synthesis of Zinc complex
An absolute EtOH solution (25 mL) of 2-pyridinecarboxaldehyde (0.22 g, 2 mmol) was added dropwise to an absolute EtOH solution (25 mL) of the appropriate diamine (0.31 g, 1 mmol). The yellow solution was stirred for 5 h; then a solution of Zn(NO3)2.6H2O (0.297 g, 1 mmol) in absolute EtOH (20 mL) was added dropwise. The solution was refluxed for 6 h, concentrated in a rotary evaporator until approximately 10–15 mL and then the complex was precipitated by addition of diethyl ether. Anal. Calcd for C31H26N6O9Zn: C, 53.81; H, 3.79; N, 12.15. Found: C, 53.93; H, 3.84; N, 12.23%. IR: 1635 (CHN)imi, 1598 (CHN)py, 1377 (NO3). 1HNMR (DMSO-d6, 300 MHz) δ 9.192 (s, 2H, CHN), 6.604–8.816 (m, 19H, aromatics), 2.389 (s, 3H, CH3) ppm. 13CNMR (DMSO-d6, 300 MHz) δ 20.524 (CH3), 163.233 (CHN), 115.330, 121.943, 125.642, 125.911, 126.576, 127.431, 128.195, 129.143, 129.729, 133.131, 137.031, 141.949, 143.822, 145.736, 145.973, 148.824, 148.990 (aromatic rings) ppm.
3.8 Synthesis of the cobalt complex
The preparation of the red complex followed the same procedure described for [ZnL(NO3)(H2O)]NO3 by using a solution of Co(NO3)2.6H2O (0.237 g, 1 mmol) in 30 mL of ethanol. Anal. Calcd for C31H24Cl2N4O2Co: C, 60.60; H, 3.94; N, 9.12. Found: C, 60.51; H, 4.05; N, 9.23%. IR: 1623 (CHN)imi, 1594 (CHN)py. 1HNMR (The 1HNMR paramagnetic spectrum of CoLCl2 was measured in the range from +120 to −120 ppm) (CDCl3, 300 MHz) δ −12.58 (s, CH3), −10.98 and −10.91 (s, CHN), 24.12, 20.11, 10.13,8.14, 7.81, 3.81, 3.26, 2.81, 2.34, 1.40 (all singlet, aromatics).
3.9 Summary of the crystallographic data for 1,2-bis(2′-nitrophenoxy)-4-methylbenzene (I)
C19H14N2O6, Mw = 366.32, monoclinic, P21/n, a = 8.1781(4), b = 15.7816(8), c = 13.7018(7) Å, β = 97.020(2)°, V = 1755.15(15) Å3, Z = 4, ρcalc = 1.386 mg/m3, T = 298 K. Reflections collected = 24994; 3082 independent reflections (Rint = 0.0383). Final R1 [for data with I > 2σ(I)] = 0.0900, wR2 = 0.2609; R1 [all data] = 0.1257, wR2 = 0.2928.
3.10 Summary of the crystallographic data for Zinc complex
C31H24N6O9Zn, Mw = 689.93, monoclinic, C2/c, a = 32.7660(12), b = 10.0461(3), c = 20.9909(10)Å, β = 116.237(3)°, V = 6197.7(4)Å3, Z = 8, ρcalc = 1.479 mg/m3, T = 298 K. Reflections collected = 171998; 5459 independent reflections (Rint = 0.0313). Final R1 [for data with I > 2σ(I)] = 0.0437, wR2 = 0.1249; R1 [all data] = 0.0515, wR2 = 0.1389.
3.11 Summary of the crystallographic data for Cobalt complex
C31H23Cl2CoN4O2, Mw = 613.36, orthorombic, Pnna, a = 14.5317(4), b = 26.9817(7), c = 14.8316(4)Å, α = β = γ = 90°, V = 5815.3(3) Å3, Z = 8, ρcalc = 1.401 mg/mm3, T = 298 K. 5713 independent reflections (Rint = 0.0720). Final R1 [for data with I > 2σ(I)] = 0.0987, wR2 = 0.2401; R1 [all data] = 0.1379, wR2 = 0.2432.
3.12 Summary computational details
All the calculations were performed with GAUSSIAN-03 packages [29]. Molecular geometries and single point energies was computed at DFT (B3LYP) [30,31] using 6-31 + G(d,p) basis set.
The first step of calculations for 1,2-bis(2′-nitrophenoxy)-4-methylbenzene (I) and 1,2-bis(2′-aminophenoxy)-4-methylbenzene (II) was performed to determine the most stable conformer.
Among the various conformers of I and II only one is a ground state. The others, while interested in and then stabilized by hydrogen bonds and intramolecular interactions, represent the transition states. The stationary structures are confirmed by ascertaining that all ground states have only real frequencies and all transition states have only one imaginary frequency. Optimized geometries are very similar to experimental one obtained by X-ray analysis.
In order to simplify the computational cost geometry optimizations and single point calculations was performed on system model built up without the 4-methyl group. On both models torsional calculations where performed starting from the most stable conformer obtained by geometric optimization and subsequently varying the twist angle α (C13O2C12C7) from the value of −40,00°. Single point energy calculations curve, reported on Schemes 2 and 3 where obtained increasing α by 5° for each step up to the α value of 140°. At this value the barrier becomes significantly high: about 30,00 Kcal/m compared to a minimum of energy observed for the value of 69.79° respectively for both α and β. From this value begins to act the strong repulsive interaction H···H (1.8104 Å) of two phenyl hydrogen atoms. Starting from this conformation was followed the trend of the PES by varying the equivalent torsion angle β. Relaxing the molecule by varying β these reach a minimum of energy, which correspond to the difference α-β and apply 9.7 and 6.9 kcal/m respectively for I and II.
As can be seen from Schemes 2 and 3, both molecules have a few rotational bonds linked to 4-methylbenzene. Among these bonds (C13O2C12C7) and (C12C7O1C6) are responsible for the flexibility and conformational stability of both compounds. Torsional flexibility around α or β dihedral angles give a great flexibility to the molecules of about 60° with a commitment energy of a few kcal. Both compounds have a high torsional barrier that prevents the complete freedom of rotation around α or β.
Acknowledgements
Support of this work by the University of Isfahan (Iran) (Grant No. 920917) is gratefully acknowledged. We would like to thank the University of Messina (Italy) for technical assistance in crystallography.