1 Introduction
Over the last decades, considerable amounts of colored wastewaters have been generated from many industries, including textile, leather, paper, printing, dyestuff, plastic, and so on [1,2]. Dye removal from contaminated water is very important because the water quality is strongly influenced by color. Even a small amount of dye is undesirable and affects dramatically the ecosystem. Moreover, many dyes employed nowadays are considered to be toxic and even carcinogenic [1,3].
Dye effluents normally contain about 10 to 50 mg/L; however, for concentrations as small as 1 mg/L, the dyes are easily noticeable and may be perceived as contaminators and consequently unacceptable. As a result, the treatments of dye effluents have been extensively investigated. Currently, the adsorption is found to be efficient because of its easy operation and the flexibility and simplicity of the design [4,5]. In this respect, activated carbon is extensively used due to its moderate price, single adsorption ability and regeneration process [6]; this explains why its use is limited. For this reason, the exploration of new and more efficient adsorption materials has important practical significance and wide application prospects for environmental protection. Cupric oxide (CuO) is a p-type semiconductor with an energy band gap of 1.21 eV, which can be excited under sunlight radiation [7]. CuO has various applications: glucose sensors [8], field emission emitters [9], gas sensors [10], and heterogeneous catalysts [11]. More recently, many studies have been focused on its application in photocatalysis. However, in most reports, CuO was used only as a sensitizer in photocatalytic heterosystems, like CuO/TiO2 [12,13], CuO/SnO2 [14], CuO/ZnO [15], and CuO/clay [16,17]. Therefore, the objective of the present work is to prepare heterogeneous a CuO catalyst supported on an NaA zeolite by exchange process and to assess its adsorption and photocatalytic activity for the degradation of methyl orange (MO) under artificial light.
2 Materials and methods
2.1 Catalyst preparation
The exchange technique was used to prepare CuO/NaA according to the following protocol: 1 g of the NaA synthesized zeolite was added to 200 mL of Cu (NO3)2·3H2O (0.1 M) (Assay, 98%) and shaken for 8 h in a polyethylene bottle at room temperature. The NaA zeolite was synthesized by hydrothermal process, as reported in our previous work [18]. The solid product was filtered and washed with doubly distilled water until Cu2+ becomes undetectable in solution. Then, the sample was dried at room temperature and referred to as CuO/NaA zeolite. Finally, CuO/NaA was calcined at 450 °C for 4 h.
2.2 Characterization
The NaA, CuO and CuO/NaA samples were characterized by X-ray diffraction (Philips PW 1800, using Cu Kα radiation), over the 2θ range between 5 and 35°. The surface morphology was observed with a scanning electronic microscope (SEM, Philips XL 30) equipped with an energy dispersive spectrometry (EDS) unit for chemical analysis.
2.3 Batch adsorption
Batch adsorption experiments of MO were carried out in a double-walled Pyrex reactor of capacity 200 cm3, whose temperature was regulated by a thermostated bath. The MO solutions (50–200 mg/L) were prepared by dilution from a stock solution (1000 mg/L) of MO (Merck, 99.5%). The effect of pH on the adsorption of MO was examined by mixing 0.2 g of CuO/NaA with 100 mL of a MO (50 mg/L) solution whose pH ranged from 3.0 to 11.0; the pH was adjusted with NaOH (0.1 N) or HCl (0.1 N). The adsorption kinetics and isotherms of the dye solutions (50–250 mg/L) were performed at different temperatures (25, 30, 40, and 55 °C). The final MO concentration was determined using a UV–Vis spectrophotometer (Optizen 2120 UV–Visible, λmax = 465 nm). The percentage of MO removal was calculated using the relationship:
(1) |
(2) |
(3) |
2.4 Photoactivity experiments
The photocatalytic degradation is undertaken with a volume of 100 mL (MO: 100 mg/L, pH ∼ 7) and 200 mg of CuO/NaA in the same reactor as above. The light source is a tungsten lamp (200 W, Philips) emitting over the range from 400 to 750 nm and located 14 cm above the reactor, thus providing a light flux of 10.3 mW·cm−2.
3 Results and discussion
3.1 Characterization
Fig. 1 shows the XRD patterns of three samples. Fig. 1a corresponds to the sodalite NaA structure with strong peaks in the 2θ range from 5 to 35°, which indicates that the zeolite was well prepared, while Fig. 1b corresponds to the CuO structure. The two phases are in good agreement with those already reported in the literature [13,18]. As expected, the XRD pattern (Fig. 1c) shows the peaks of the zeolite NaA, which coexist with those of CuO.
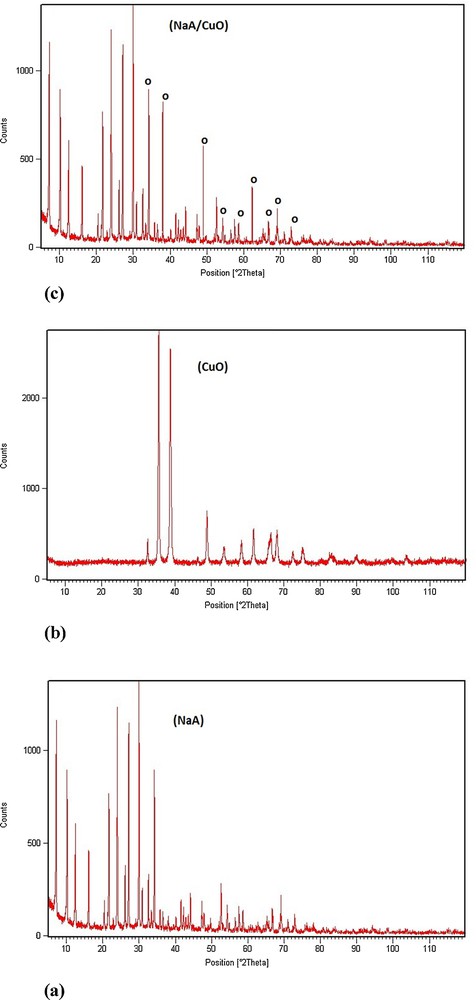
XRD patterns of NaA (a), CuO (b) and CuO/NaA (c).
Scanning electronic micrographs (Fig. 2a) show that the crystallites of an NaA zeolite form fine cubic particles with an average size of 2–5 μm. Fig. 2b shows that CuO particles crystallize as needles, with an average size of about 0.1–0.5 μm, and form spherical aggregates. It seems that the preparation method of this oxide has a positive influence on crystal morphology. Fig. 2c shows that CuO particles are well dispersed in the structure surface of the NaA zeolite. The chemical analysis by EDS confirmed the presence of copper on the surface of the NaA zeolite.
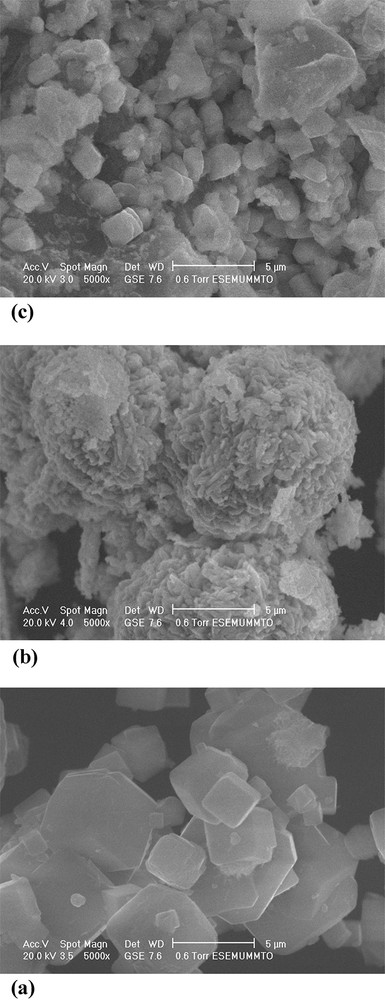
SEM images of the zeolite NaA (a), CuO (b) and CuO/NaA (c).
3.2 Adsorption
3.2.1 Effect of pH
The pH of the solution is a controlling parameter that strongly affects the adsorption of dyes on the CuO/NaA surface. The influence of pH on MO adsorption was investigated over the range from 3 to 11 and the results are displayed in Fig. 3, which shows a maximum removal of MO (99%) at pH ∼ 7. At lower pHs values, the adsorbants have a net positive charge. Hence, the minimum MO removal obtained at low pH may be due to the electrostatic attractions between negatively charged functional groups located on MO and the positively charged adsorbent surface. Hydrogen ion also acts as a bridging ligand between the adsorbent and dye molecules [20]. As pH increases, the number of hydroxide ions increases and competes with anionic ion MO on the adsorption. A similar type of behavior was reported for the adsorption of the dye onto different adsorbents [20]. Therefore, a pH value ∼ 7 was selected for further studies.
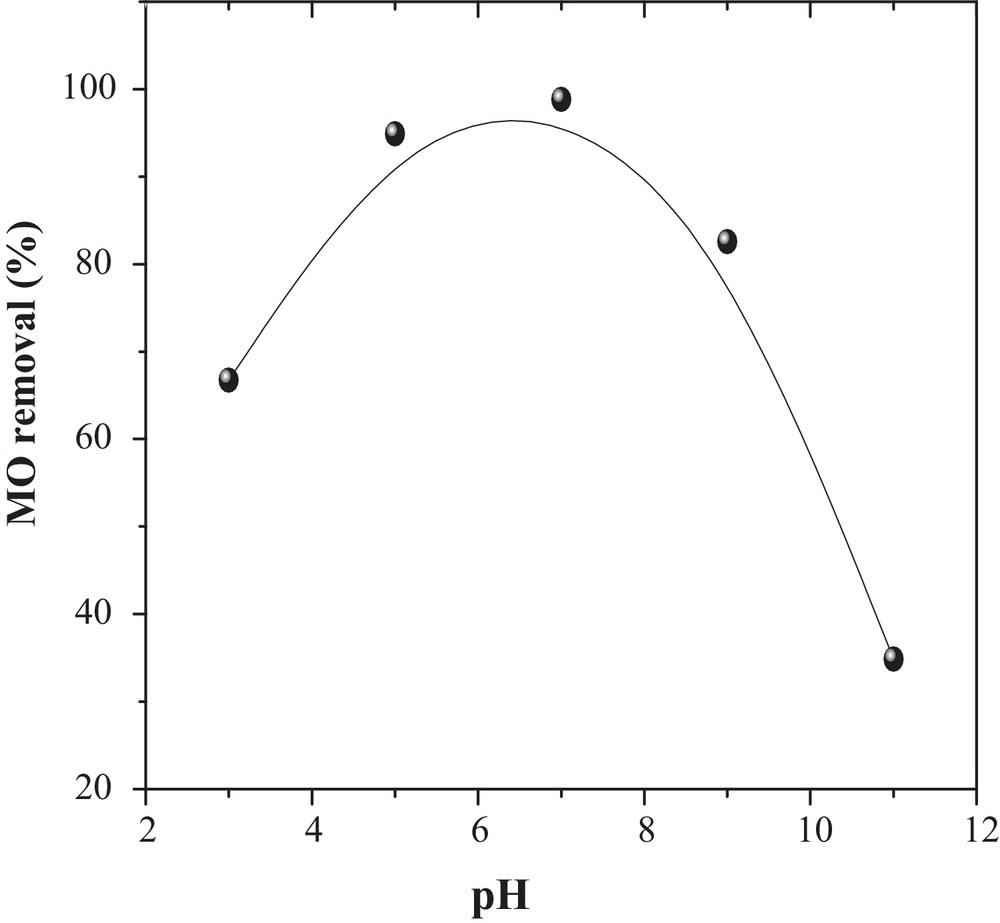
Effect of pH on the removal of MO onto CuO/NaA (MO = 50 mg/L, catalyst dose = 2 g/L, T = 298 K and time of agitation = 5 h).
3.2.2 Effect of contact time
The effect of the contact time on the removal percentage of MO was investigated for a concentration of 50 mg/L (Fig. 4). The uptake of MO by CuO/NaA was rapid at the beginning, due to a larger surface area of adsorbent, and gradually decreases with time until it reaches saturation. The plots reveal that the maximum removal is achieved after 240 min of shaking. After adsorption, MO uptake is controlled by the rate of dye transported from the exterior to the internal pores of the adsorbent.
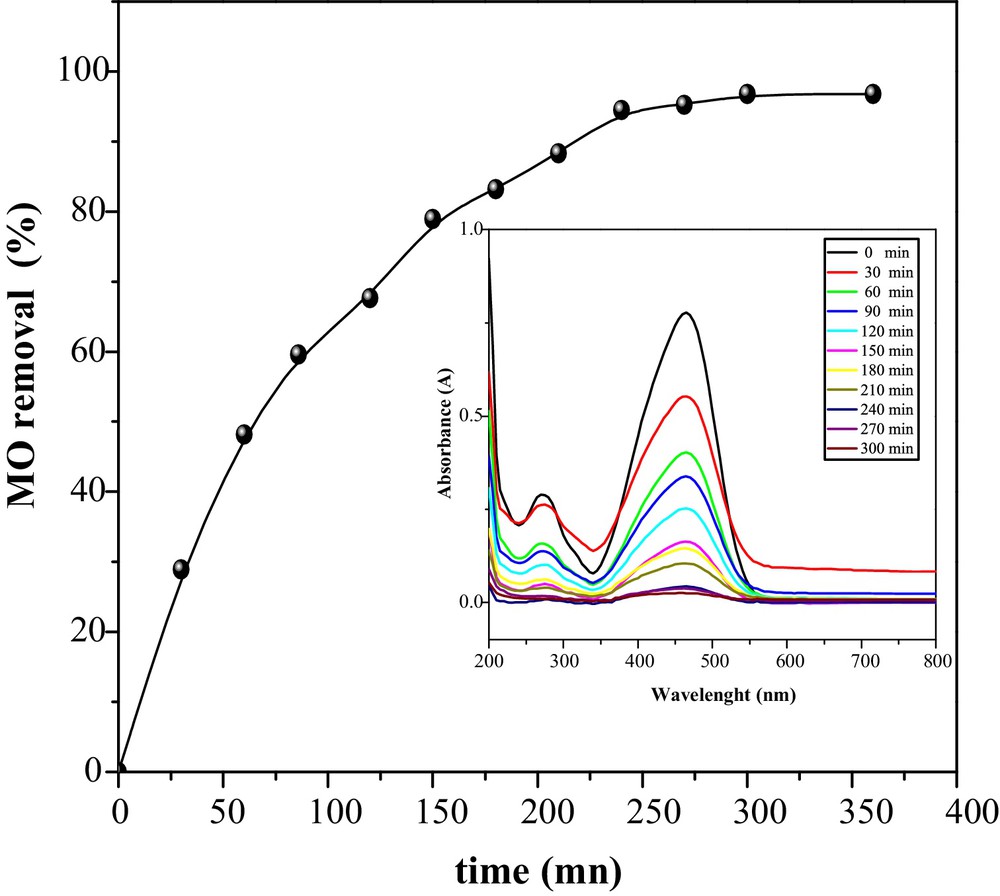
Time of contact on the removal of MO onto CuO/NaA (MO = 50 mg/L, pH ∼ 7, catalyst dose = 2 g/L and T = 298 K).
3.2.3 Effect of the catalyst dose
The adsorbent dose is an important parameter since it determines the uptake capacity of an adsorbent for a given initial MO concentration under the operating conditions. The influence of the dose on MO adsorption (Fig. 5) increases with increasing the dose of CuO/NaA, and the adsorption is almost constant at doses higher than 2 g/L. This may be due to the increased availability of surface active sites resulting from the increased dose and conglomeration of the adsorbent.
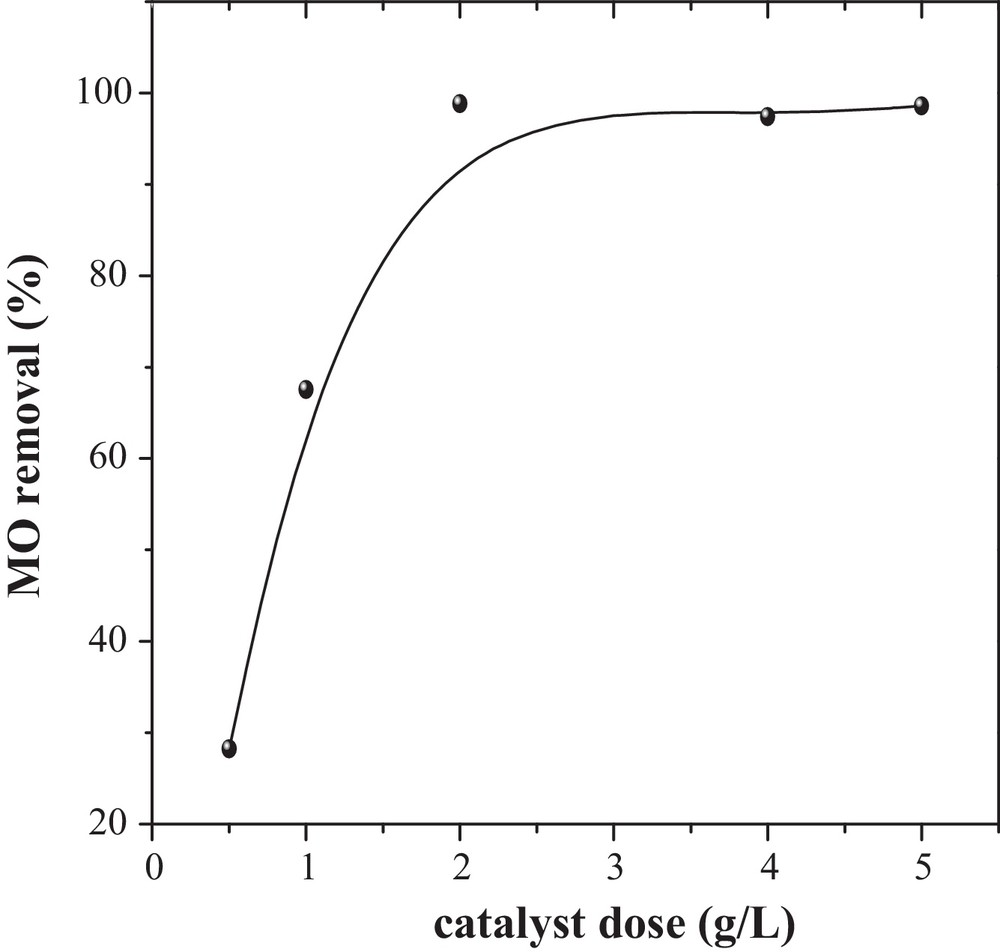
Effect of the dose of catalyst on the removal of MO onto CuO/NaA (MO = 50 mg/L, pH ∼ 7, T = 298 K).
3.2.4 Effect of initial concentration of MO
The effect of MO concentration over the range (50–250 mg/L) was investigated with a dose of 2 g/L, pH ∼ 7, a temperature of 25 °C and a duration of 6 h. The results show that the percentage removal of MO was found to decrease with increasing the initial MO concentration, achieving a maximum removal of 97% at 50 mg/L and of 60% for 250 mg/L (Fig. 6). This strong adsorption for low initial MO concentrations might be explained by the active sites available on the adsorbent. Similar results have been reported in the literature [21–23].
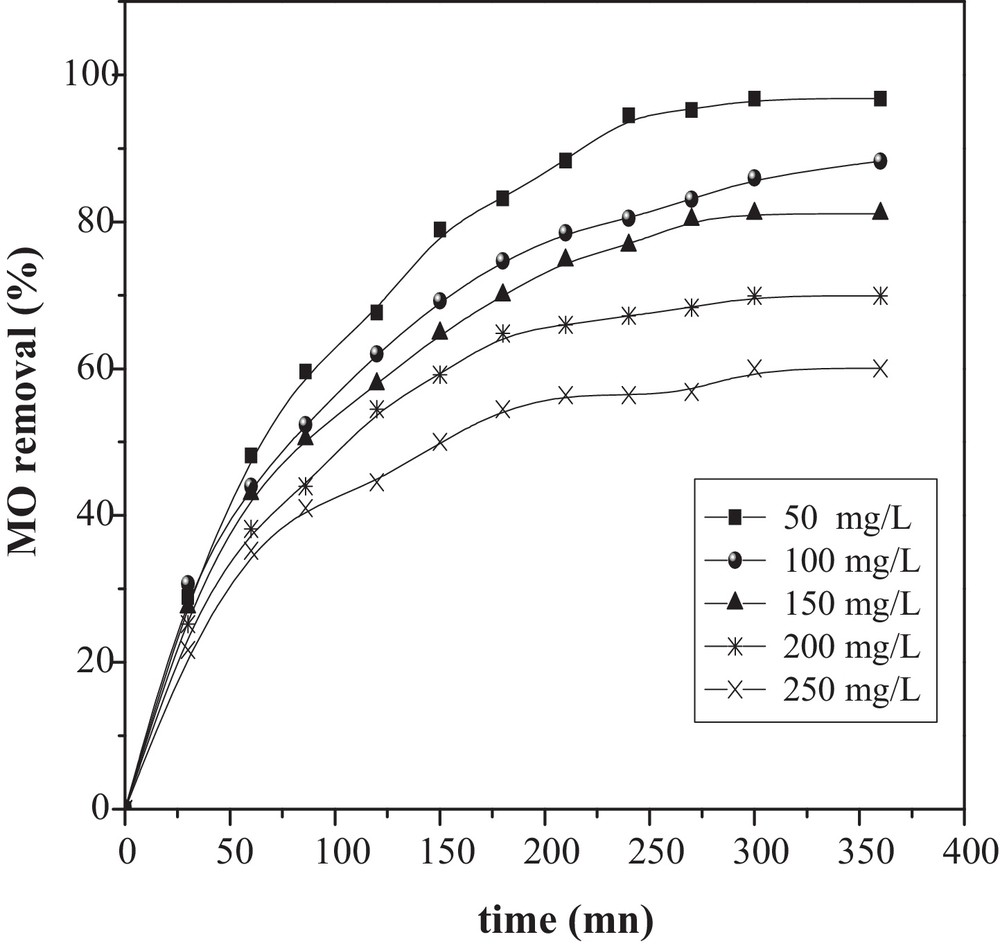
Effect of initial concentration and contact time on the removal of MO onto CuO/NaA. (pH ∼ 7, catalyst dose = 2 g/L and T = 298 K).
3.2.5 Effect of temperature
The adsorption of MO onto CuO/NaA was studied for a concentration of 50 mg/L by varying the temperature from 25 to 55 °C (Fig. 7). The percentage of MO removal increased with increasing the temperature from 25 to 55 °C; it can be seen that higher temperatures were favorable for adsorption. The equilibrium adsorption capacity was affected by the temperature and the amount of MO adsorbed increased from 67.55 to 94% when the temperature was raised from 25 to 55 °C. It has been well documented that the temperature has two major effects on the adsorption process. An increase in temperature is known to increase the diffusion rate of the adsorbate molecules across the external boundary layer and in the internal pores of the adsorbent particles as a result of the decreased viscosity of the solution.
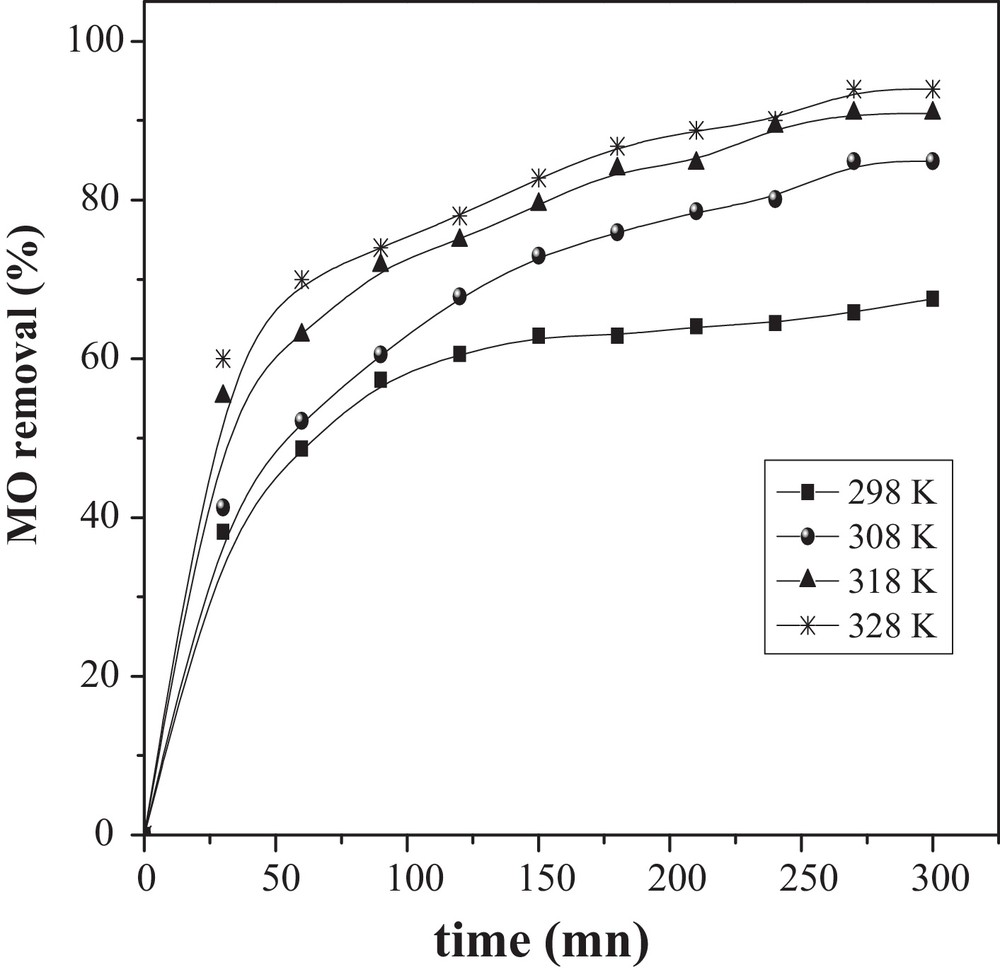
Effect of temperature and contact time on the removal of MO by CuO/NaA (MO = 50 mg/L, pH ∼ 7 and catalyst dose = 1 g/L).
3.3 Adsorption isotherms
In order to describe the removal mechanism of MO from water onto CuO/NaA, three isotherm models (Freundlich, Langmuir and Temkin isotherm models) were applied. The Langmuir, Freundlich and Temkin isotherm models were applied to establish the relationship between the amount of MO adsorbed by CuO/NaA and their equilibrium concentration in aqueous solution. The experimental data are fitted by the Langmuir model [24]:
(4) |
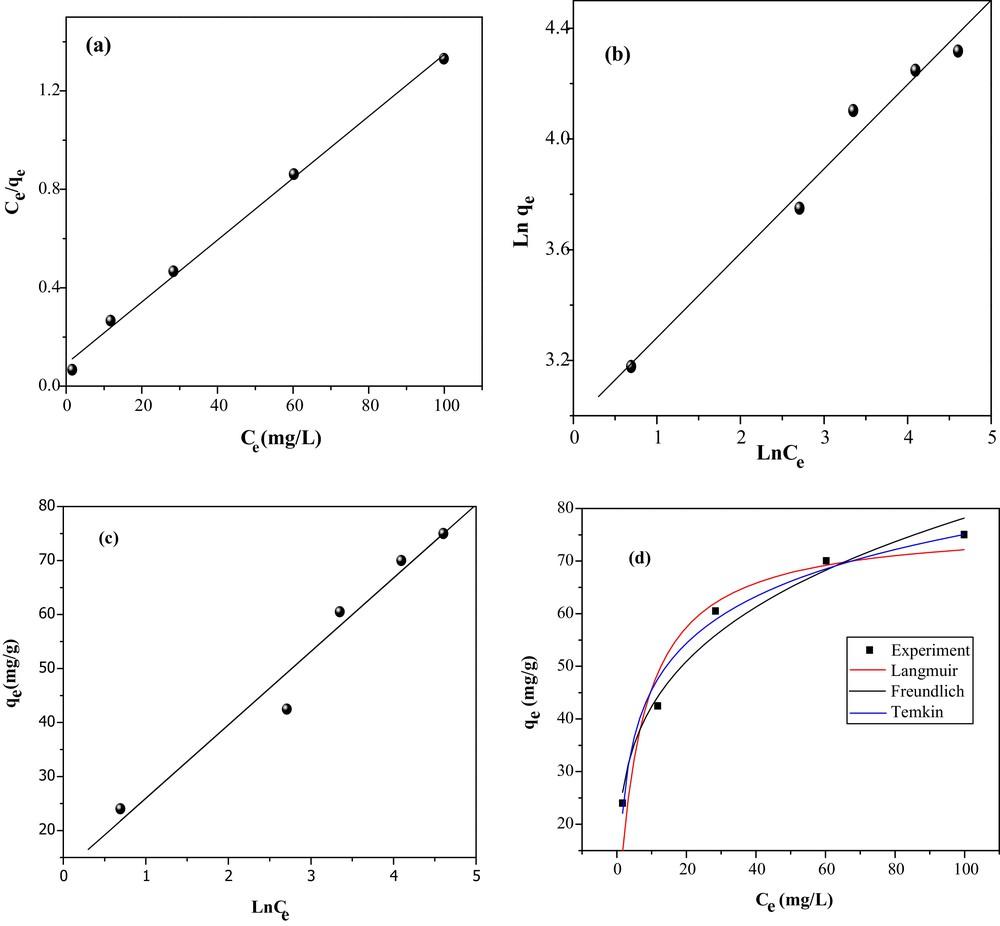
(a) Langmuir, (b) Freudlich, (c) Temkin isotherms for the MO adsorption and experimental data that do not arrange linearly.
Comparison of the equilibrium isotherm models.
Langmuir | |
qm (mg/g) | 79.49 |
b (L/mg) | 0.108 |
R2 | 0.995 |
Freundlich | |
KF (mg/g) | 19.636 |
n | 3.28 |
R2 | 0.979 |
Temkin | |
bT (J/mol) | 182.317 |
AT (L/g) | 2.492 |
R2 | 0.955 |
The adsorption equilibrium data were also applied to the Freundlich model in logarithmic form [25]:
(5) |
The Temkin isotherm contains a factor that explicitly takes into account adsorbent–adsorbate interactions. By ignoring the extremely low and large concentrations, the model assumes that the heat of adsorption (function of temperature) of all molecules in the layer would decrease linearly rather than logarithmically with coverage. The derivation is characterized by a uniform distribution of binding energies (up to some maximum binding energy) that was evidenced by plotting qe against ln Ce [Eq. (6)]:
(6) |
where AT (L/g) and B (J/mol) are the Temkin constants related to the binding equilibrium isotherm and to the heat of adsorption, respectively. The constants were determined from the slope and the intercept.
The adsorption of MO onto CuO/NaA was well fitted with the Langmuir, Freundlich and Temkin models because of the high correlation coefficients (R2). The Langmuir model (R2 = 0.995) was more applicable than those of Freundlich (R2 = 0.979) and Temkin (R2 = 0.955). The Langmuir adsorption maximum, qm, is quite high (79.49 mg/g), with a constant b of 0.108 L/mg. The Freundlich coefficient (n = 3.28) was smaller than 10, indicating that the adsorption of MO on CuO/NaA under the studied conditions was favored. By contrast, Fig. 8 shows experimental data that do not arrange linearly. It seems that the data are well fitted by using the models of Langmuir and Temkin.
3.4 Adsorption kinetics
The adsorption kinetics is important because it controls the efficiency of the process and the equilibrium time. It also describes the rate of adsorbate uptake on CuO/NaA. In order to identify the potential rate-controlling steps involved in the adsorption process, two kinetic models were used to fit the experimental data, namely the pseudo-first-order and pseudo-second-order models.
3.4.1 Lagergren pseudo-first-order model
The Lagergren equation is the earliest known example describing the rate of adsorption in the liquid–phase systems; it involves a pseudo-first-order kinetics [26,28]:
(7) |
(8) |
The values of k1 can be obtained from the slope of the linear plot of versus t.
3.4.2 Pseudo-second-order model
The second-order kinetics may be tested on the basis of the following equation [29]:
(9) |
(10) |
The plots of t/qt versus time (t) for different concentrations (Co) and various temperatures fit well with the experimental data and provide the rate constants k2 and qe. Plots of Lagergren first-order and pseudo-second-order kinetic models are shown in Figs. 9 and 10 for different MO concentrations and temperatures (Table 2). The R2 coefficients of the pseudo-second-order kinetics were higher than 0.994 for both solutions. Moreover, the calculated qe value agrees well with the experimental one, indicating that the model fits better the adsorption data.
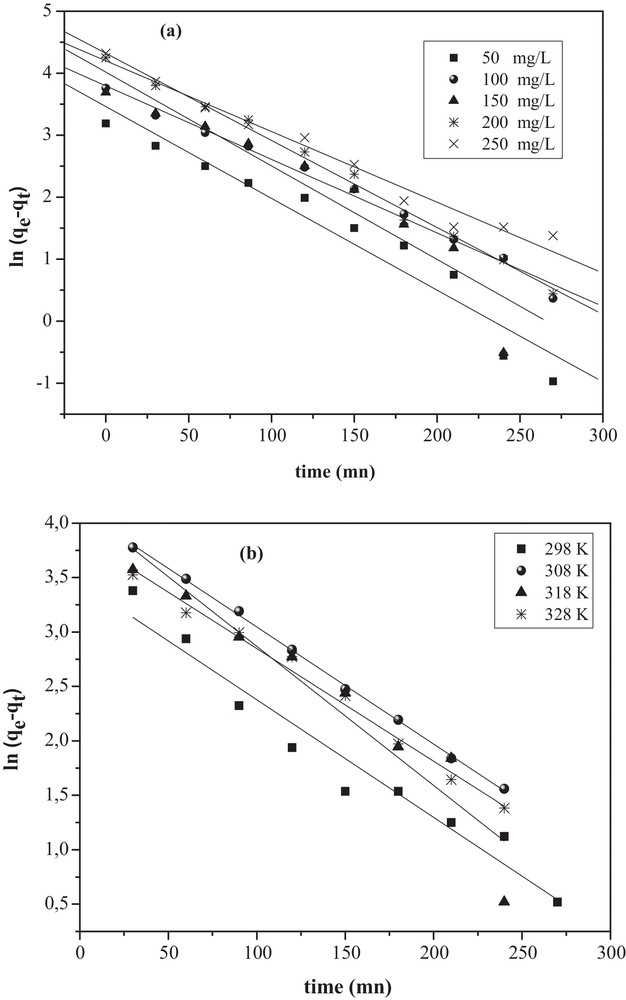
Pseudo-first-order kinetic model for the removal of MO onto CuO/NaA under: (a) different initial concentrations (Co); (b) different temperatures.
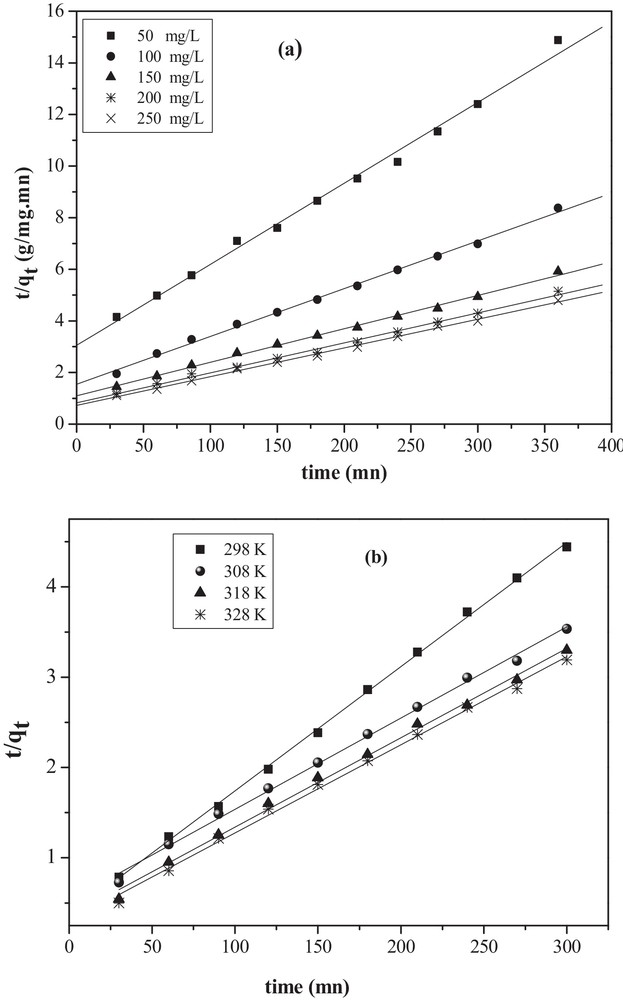
Pseudo-second-order kinetic model for the removal of MO onto CuO/NaA under: (a) different initial concentrations (Co); (b) different temperatures.
Pseudo-first-order and pseudo-second-order models constants for the adsorption of MO on CuO/NaA under different initial concentrations and different temperatures.
Pseudo-first-order | Pseudo-second-order | |||||
qecal (mg/g) | k1 × 102 (min−1) | R2 | qecal (mg/g) | k2 × 104 (g·mg−1·min−1) | R2 | |
MO (mg/L) | ||||||
50 | 31.74 | 1.48 | 0.941 | 31.84 | 3.22 | 0.994 |
100 | 44.53 | 1.19 | 0.990 | 54.05 | 2.21 | 0.997 |
150 | 55.49 | 1.51 | 0.899 | 76.92 | 1.57 | 0.996 |
200 | 75.07 | 1.40 | 0.992 | 86.20 | 1.62 | 0.995 |
250 | 66.63 | 1.14 | 0.975 | 90.09 | 1.69 | 0.997 |
T (K) | ||||||
298 | 31.76 | 1.08 | 0.9458 | 72.67 | 5.18 | 0.9989 |
308 | 61.72 | 1.07 | 0.9988 | 98.91 | 1.94 | 0.9965 |
318 | 63.25 | 1.28 | 0.9006 | 101.01 | 2.81 | 0.9961 |
328 | 48.58 | 1.03 | 0.9879 | 102.46 | 3.19 | 0.9962 |
The rate constants k2 at different temperatures (Table 2) were used to estimate the activation energy of MO adsorption onto CuO/NaA. The relationship among the rate constant k2, temperature (T) and activation energy (Ea) follows the Arrhenius equation [30]:
(11) |
the linearization gives:
(12) |
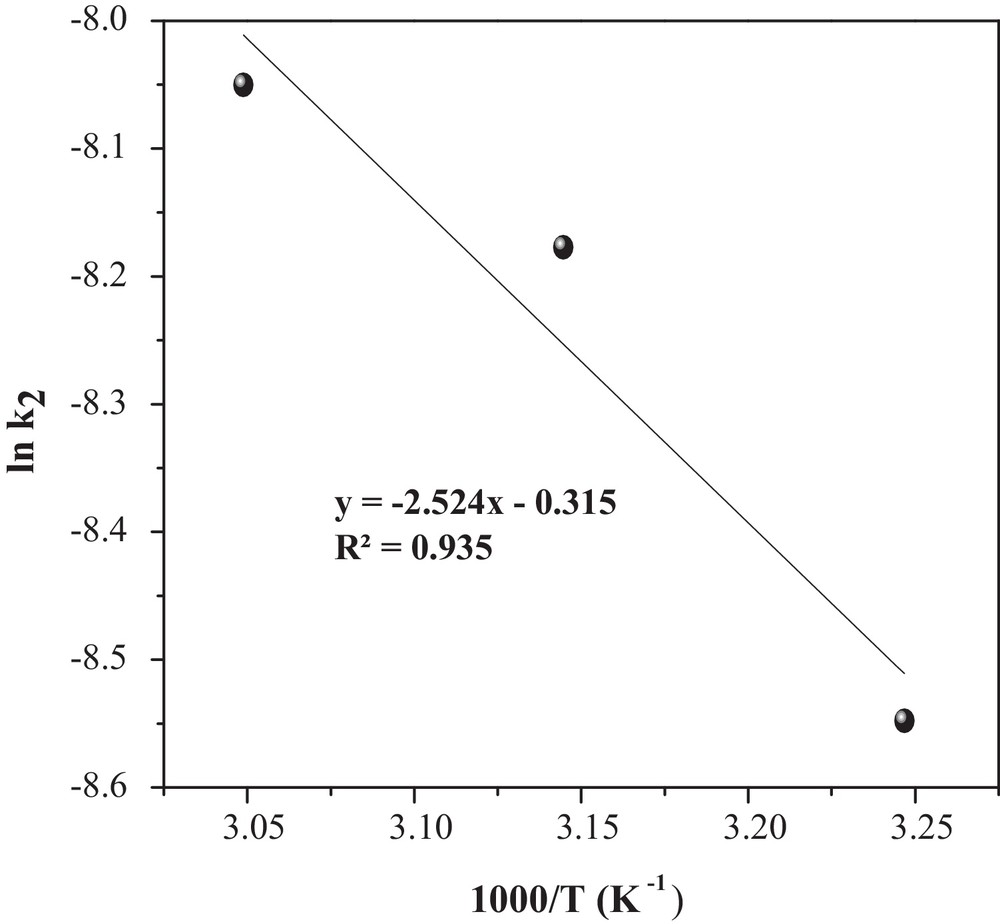
Determination of the adsorption activation energy (Ea).
3.5 Thermodynamic study
The thermodynamic parameters were evaluated to confirm the nature of the adsorption and the inherent energetic changes involved during MO adsorption. Standard enthalpy (ΔH°), free energy (ΔG°) and entropy change (ΔS°) were calculated to determine the thermodynamic feasibility and the spontaneous nature of the process. Therefore, the values of ΔH° and ΔS° were obtained from the slope and intercept of the ln Kd versus 1/T curve according to Eq. (12) (Fig. 12) [18]:
(12) |
ΔG° = ΔH° – TΔS° | (13) |
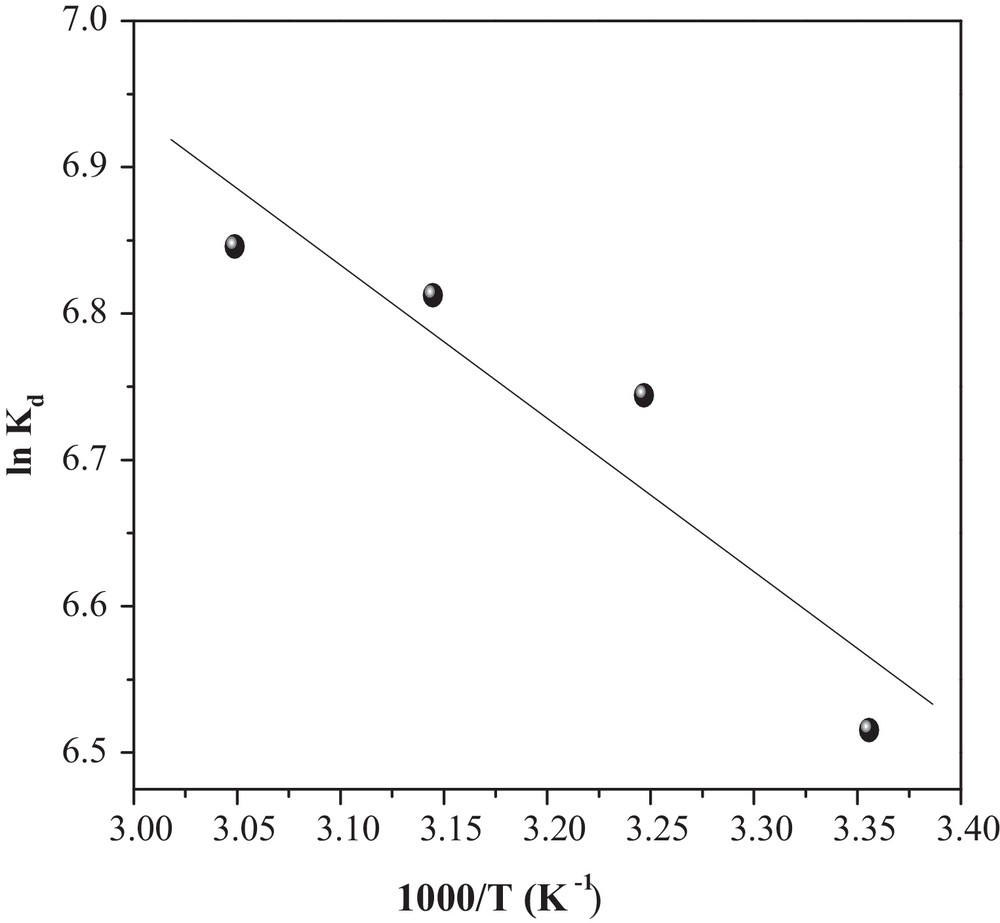
Graphical determination of ΔH° and ΔS°.
As can be seen in Table 3, the ΔG° values are negative for all temperatures, indicating that MO adsorbed spontaneously onto CuO/NaA and that the system does not gain energy from an external source. The positive value of ΔH° further confirms the endothermic nature of MO adsorption, while positive entropy (ΔS°) indicates the increased randomness with MO adsorption, probably because the number of desorbed water molecules is larger than that of adsorbed MO molecules.
Thermodynamic parameters for the adsorption of MO onto CuO/NaA at different temperatures (MO = 50 mg/L, pH ∼ 7, dose of catalyst = 1 g/L).
T (K) | Thermodynamic parameters | ||
ΔG° (kJ/mol) | ΔH° (kJ/mol) | ΔS° (J/mol K) | |
298 | –16.265 | ||
308 | –17.103 | 8.705 | 83.797 |
318 | –17.941 | ||
328 | –18.779 |
3.6 Photocatalysis
It is worthwhile to outline that MO (with a concentration of 100 mg/L) was partially adsorbed (88%). So, the remaining concentration was subjected to visible illumination. The kinetic was studied by withdrawing aliquots at regular time intervals. Fig. 13 clearly shows that MO photodegradation obeys a first-order kinetic with a rate constant of 7.47 ×10−3 min−1 and a removal percentage of 76%.
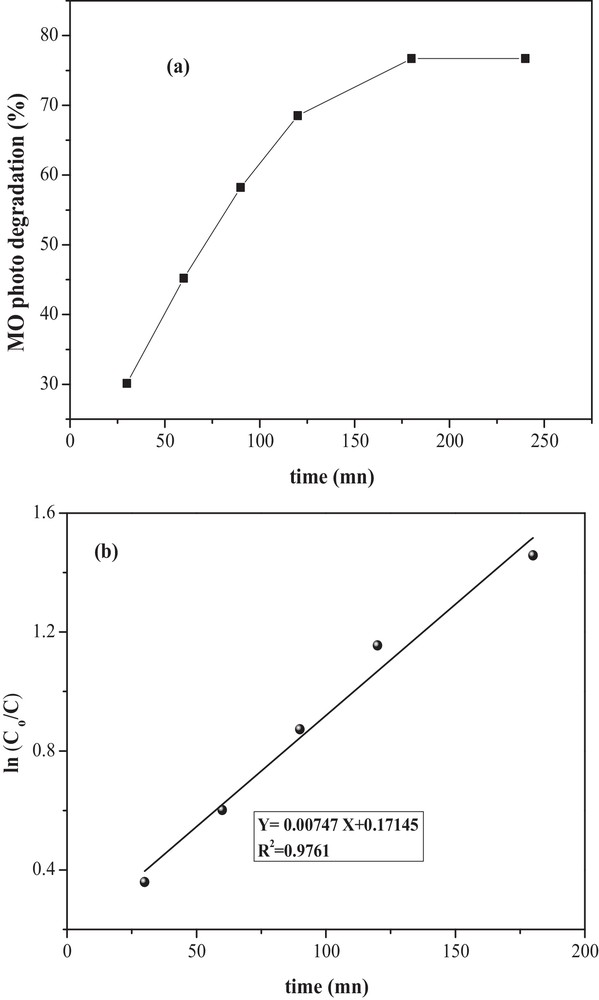
a: MO photodegradation by CuO/NaA; b: photocatalytic degradation kinetic of MO (MO = 12 mg/L, pH ∼ 7, catalyst dose = 2 g/L and T = 298 K).
From Table 4, comparing the maximum adsorption capacities qm (mg/g) of MO on different adsorbents, shows that the CuO supported on NaA zeolite performs considerably better than the other ones.
Comparison of maximum adsorption capacities qm (mg/g) of MO onto different adsorbents.
Adsorbent | qm (mg/g) | Reference |
γ-Fe2O3/SiO2/CS composite | 34.29 | [1] |
CuO/NaA zeolite | 79.49 | This study |
Carbon coated monolith | 27.2 | [5] |
Hypercrosslinked polymeric | 76.92 | [25] |
Multiwalled carbon nanotubes | 52.86 | [27] |
Natural zeolite modified with hexamethylenediamine | 33.0 | [28] |
Surfactant modified silkworm exuviae | 87.03 | [31] |
De-oiled soya | 13.46 | [32] |
4 Conclusion
CuO supported on a NaA zeolite was elaborated by an exchange method and characterized by X-ray diffraction and scanning electron microscopy. The physical parameters of the adsorption of methyl orange, like the solution pH, the dose of catalyst, the MO concentration and temperature were optimized. The data were well fitted by the Langmuir model. The disappearance of MO follows a second-order kinetic, and the thermodynamic parameters indicate a spontaneous and endothermic process. The catalyst CuO/NaA has been successfully tested for the photodegradation of methyl orange under visible light.