1 Introduction
Since Jean-Pierre Sauvage reported in 1983 the first templated synthesis of a [2]catenane [1], considerable research efforts have been devoted to the development of novel synthetic strategies allowing the efficient preparation of interlocked molecular architectures [2–4]. Forty years of investigations in this field gave birth to a broad collection of structurally diverse rotaxanes, catenanes and knots exhibiting unique chemical properties. More recently, the functional aspects of such interlocked systems has become the subject of intense research to find practical applications of mechanically bonded molecules [5]. Within this framework, rotaxanes have been widely studied as potential electronic devices, materials, sensors and key components for molecular motors and machines [6–13]. Furthermore, some rotaxane- and pseudorotaxane-based devices have been designed to interact with biological systems, especially for the delivery of bioactive compounds or biomedical imaging. In this short review, we report the advances that have been realized in this burgeoning area of research over the past 15 years. Herein, we will summarize successively the main advances made in the design of squaraine-rotaxane dyes for optical bioimaging applications, host-rotaxanes as cellular transport agents, enzyme-responsive rotaxanes and mechanized mesoporous silica nanoparticles coated with “nanovalves” as drug delivery systems.
2 Squaraine-rotaxane dyes for optical bioimaging applications
Squaraines are a class of near-IR dyes with promising photophysical properties for optical bioimaging applications [14]. However, their use as fluorescent probes is limited by their inherent chemical instability that results from the attack of biological nucleophiles on the electron-deficient cyclobutene ring. Additionally, squaraines readily form aggregates in water which quench fluorescence. To circumvent these drawbacks, Smith et al. proposed to encapsulate the cyclobutene core of the squaraine dye as the thread component inside an interlocked rotaxane structure [15–17]. Thus, using the hydrogen bond directed clipping reaction developed earlier by Leigh et al. [18–20], they synthesized a range of squaraine-derived rotaxanes such as 1 (Scheme 1) [21–22].

Squaraine-rotaxane dyes for optical bioimaging applications.
As expected, the encapsulating macrocycle inhibited aggregation and greatly enhanced the stability of the squaraine dye in various media, preventing nucleophilic attack on the cyclobutene moiety. Therefore, when incubated with bovine serum, rotaxane 1a was stable for many weeks whereas the corresponding thread was degraded over a few minutes [17].
Soon after, Smith et al. published a remarkable extension of this work where they compared the performance advantages of rotaxane 1b over the commonly used sulfonated cyanine dye Cy-5 [23]. Rotaxane 1b includes zinc(II)-dipicolyl-amine (Zn-DPA) stoppers that also play the role of targeting ligands since Zn-DPA groups are known to target the anionic surfaces of bacterial cells [24]. The high stability of 1b permitted real time fluorescence-imaging of Escherichia coli bacteria undergoing cell division, which was not possible using probes based on Cy-5. Futhermore, rotaxane 1b allowed optical imaging of Staphylococcus aureus and E. coli bacteria in living nude mice showing the great potential of squaraine-rotaxane dyes as bioimaging agents.
The same group developed the highly innovative interlocked system 2 that may be suitable for many types of molecular imaging and biotechnology applications (Scheme 2) [25].

A squaraine-rotaxane dye for in vivo dual-modality molecular imaging.
Rotaxane 2 that features an endoperoxide macrocycle was easily prepared from 3 by oxidation of one anthracene unit in the presence of air under irradiation with red light. The synthesis of precursor 3 was achieved using the “Clicked Capping” method reported earlier by Smith et al. [26,27]. With this design, interlocked system 2 was very stable and can be stored at −20 °C during extremely long periods without any degradation. However, upon warming at 37 °C, compound 2 underwent a unimolecular cycloreversion reaction leading to the release of singlet oxygen together with the emission of near-IR light. Thus, such a thermally activated rotaxane-based dye possesses both chemiluminescence and fluorescence properties making this interlocked molecular system particularly interesting for optical imaging. The validity of this concept was evaluated in living mouse with 2-labelled microparticles [25]. The results obtained in this study demonstrated that squaraine rotaxanes including an endoperoxide macrocycle can be used in vivo as dual-modality molecular imaging probes.
3 Host-rotaxanes as cellular transport agents
In 2003, Smithrud et al. proposed the use of host-[2]rotaxanes with the aim to create synthetic compounds that mimic proteins ensuring the functions of cellular transport agents [28]. For this purpose, they designed a series of [2]rotaxanes 4 containing an arginine-derivatized dibenzyl-24-crown-8 (DB24C8) and a number of host blocking groups, allowing the encapsulation of various guest molecules (Scheme 3) [29]. With this design, the wheel can freely slide along the thread, therefore adjusting its position to form the most stable conformation for the recognition of a given guest [30]. Also, the nature of the host blocking group can be easily modulated by the DCC-rotaxane method developed by the same group [31].

Host-rotaxanes as cellular transport agents.
Rotaxanes 4a and 4b, including an aromatic cleft and a cyclophane pocket, respectively, were studied for the delivery of peptides into cells (Scheme 3) [32,33]. Indeed, while many bioactive peptides hold great promise as therapeutic agents, their use in vivo is hampered by poor bioavailability mainly due to their inability to pass through cellular membranes. Investigations conducted with 4a confirmed the importance of conformational flexibility in the ability of host-[2]rotaxanes to bind guests and transport peptides into cells. Thus, Smithrud et al. demonstrated that pre-incubation of rotaxane 4a with COS-7 cells significantly increased the uptake of a fluoresceinated pentapeptide (AVWAL). Furthermore, since cellular uptake was largely temperature- and ATP-independent, it was suggested that the rotaxane-peptide non-covalent complex passed through the cell membrane via a passive mechanism. More recently, the same authors developed an analogue of 4a containing an oligo(ethylene glycol) chain on the axle, thereby increasing the length of the latter [34]. Interestingly, this new host-[2]rotaxane delivered more efficiently a range of fluoresceinated peptides into ovarian tumour cells than 4a which possesses a shorter axle.
In a comparative study, Smithrud et al. showed that the ability of cyclophane-[2]rotaxane 4b to transport fluorescein into COS-7 cells was substantially better than that of the cleft-[2]rotaxane 4a [28]. Additionally, 4b transported fluorescein to a greater extent into the nucleus, whereas 4a delivered the fluorescent probe more uniformly throughout a cell. As the cyclophane pocket appeared to be beneficial for cell transport, rotaxane 4b was tested for the intracellular delivery of peptides possessing a wide range of side chains (displaying apolar and polar groups either negatively or positively charged). These experiments demonstrated the utility of rotaxane 4b for the transport of peptides of all polarity across cell membranes, highlighting the versatility of this concept.
More recently, Smithrud et al. studied host-[2]rotaxanes enabling the transfer of alkali and alkaline cations from aqueous solutions into chloroform [35]. In this context, they proposed the use of the crown ether host-rotaxanes 5 as novel bacterial ionophores (Scheme 4) [36]. The authors hypothesized that rotaxanes 5 would increase the intracellular concentration of metal ions such as Ca2+ and Mg2+, thereby inducing cell death through apoptosis. When incubated with SKOV-3 ovarian cancer cells, host-rotaxanes 5a and 5b were cytotoxic in the low micromolar range. The cytotoxicity of rotaxanes increased with the concentration of either Ca2+ or Mg2+ confirming that 5a and 5b operated as expected by enhancing the intracellular concentration of these ions.

Host-rotaxanes as bacterial ionophores.
4 Enzyme-responsive rotaxanes
Besides their poor ability to cross cell membranes, many bioactive peptides suffer from in vivo instability as a result of rapid enzymatic degradation. For instance, Met-enkephalin is a pentapeptide (YGGFM) with interesting antiproliferative activities against several cancer cell lines [37–39]. However, this compound cannot be used as an anticancer drug since it is degraded in less than 5 min in human plasma. With the aim to circumvent this drawback, our group, in collaboration with those of Leigh and Aucagne, proposed the concept of enzyme-responsive rotaxane-based propeptides [40]. Within this framework, a pilot study was conducted with the glycorotaxane [41–44] 6 programmed for the selective delivery of Met-enkephalin at the tumour site (Scheme 5). This mechanically interlocked system was composed of a galactoside trigger, a self-immolative stopper [45,46], a pentapeptide-based axle and a benzylic amide macrocycle [18,47,48]. With this design, we hypothesized that the macrocycle should play the role of a temporary molecular shield protecting the peptidic bonds of Met-enkephalin from degradation by plasmatic peptidases. Thus, when incubated in human plasma containing peptidases responsible of Met-enkephalin degradation, rotaxane 6 was perfectly stable over several days. In contrast, the corresponding thread was degraded with a half-life of 5 h under the same conditions, therefore demonstrating that encapsulation of the pentapeptide within the rotaxane structure 6 prevented its peptidase-mediated hydrolysis in plasma. We also showed that the active peptide can be readily released in the presence of β-galactosidase, an enzyme that has been successfully targeted [49] in tumour tissues via antibody-directed enzyme prodrug therapy strategies (ADEPT [50,51]). As illustrated in Scheme 5, activation of the galactoside trigger led to the formation of an unstable phenolate intermediate that underwent a 1,6-elimination followed by a spontaneous decarboxylation, enabling the disassembly of the mechanically interlocked components and the subsequent liberation of the free bioactive pentapeptide.

Met-enkephalin rotaxane propeptide.
While our study confirmed the potential of this approach for the transport and delivery of peptides with antitumour activity, rotaxane 6 exhibited poor aqueous solubility. We then decided to pursue our investigations in this field with the galactosidase-responsive rotaxane propeptides 7 composed of a novel self-immolative linker and a triglycyl peptide encapsulated by a macrocycle bearing two hydrophilic moieties (Scheme 6) [52]. The compounds 7a and 7b were easily prepared via post-functionalisation of the same bis-azido rotaxane 7c using the well-known CuAAC ‘click’ reaction. Under such circumstances, the clickable precursor 7c appeared as a useful platform for accessing a wide range of propeptides decorated for specific biological applications. As expected both rotaxanes 7a and 7b were more soluble in aqueous media than the undecorated rotaxane analogue 7d. Interestingly, the introduction of the two glucosylated tetra(ethylene glycol) substituents (rotaxane 7b) afforded an excellent aqueous solubility that was 50,000-fold higher than that of rotaxane 7d. Moreover, the presence of the bulky hydrophilic groups on the macrocycle did not alter the recognition of the galactoside trigger, as the activation of the rotaxane propeptide 7b with β-galactosidase efficiently released the parent tripeptide.

Rotaxane-based propeptides.
More recently, our group developed the biocompatible [2]rotaxane 8 programmed for the intracellular delivery of paclitaxel while preventing its premature liberation in plasma (Scheme 7) [53]. To achieve such a task in an autonomous manner, the interlocked molecular system 8 includes an enzymatic trigger, a self-immolative linker, a self-opening macrocycle, a hydrophilic stopper and an esterase-sensitive thread linked to the C2′-OH position of paclitaxel. In the blood stream, the macrocycle acts as a molecular shield protecting the ester bond from plasmatic esterase-mediated hydrolysis. However, in the presence of β-galactosidase, the cleavage of the galactoside trigger initiates the disassembly of the mechanically interlocked components, leading ultimately to the liberation of the free axle as illustrated in Scheme 7. Unmasked in this way, the C2′-OH ester of paclitaxel becomes accessible to esterases which can therefore induce the release of the drug. As the activity β-galactosidase is mainly intracellular, the decomposition of rotaxane 8 occurs exclusively within living cells. Moreover, this interlocked functional system exhibits an appreciable level of selectivity for cancer cells overexpressing β-galactosidase which is of interest since the selective killing of tumour cells represents one of the main challenges of cancer chemotherapy.
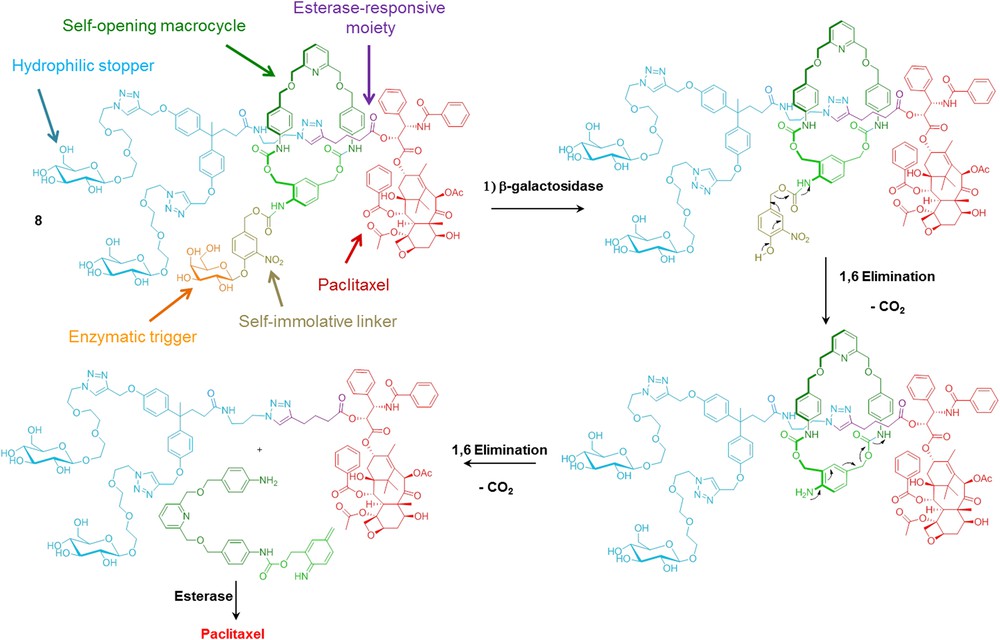
An enzyme-responsive rotaxane with a self-opening macrocycle.
5 Rotaxane- and pseudorotaxane-based ‘nanovalves’
In 2005, Stoddart and Zink were the first to propose the use of mechanically interlocked molecules as ‘nanovalves’ for mechanized silica nanoparticles [54]. Mesoporous silica nanoparticles (MSNPs) possess the ability to encapsulate a wide variety of organic molecules and can accumulate passively in solid tumours (the enhanced permeability and retention of macromolecules in solid tumours is known as the EPR effect [55]). As a result, MSNPs have been considered as potential carriers for the delivery of therapeutic and diagnostic agents [56,57]. By attaching either rotaxane or pseudorotaxane architectures to the silica matrix, Stoddart and Zink aimed to release host molecules in a stringently controlled fashion using a mobile macrocycle as a valve. In this approach, when the macrocycle is close to the surface of the particle (valves ‘closed’), the guest molecules remain trapped within the pores of silica. On the other hand, in response to a specific stimulus, the macrocycle can move away from the surface (valves ‘opened’), thereby allowing the liberation of cargo molecules. Based on this principle, several types of ‘nanovalves’ have been studied in order to control the nanopore opening using various stimuli such as light, oxidation/reduction, changes in pH, and enzymes [51,58–66]. Until now, most of these functional systems have been designed to operate exclusively under abiotic conditions. However, a few remarkable examples of MSNPs functionalized with ‘nanovalves’, capable of delivering potent anticancer drugs autonomously within living tumour cells in vitro and in vivo, have been recently reported in the literature [67,68]. In this context, the first example is the pH-sensitive supramolecular ‘nanovalve’ 9 composed of an N-methylbenzimidazole stalk attached covalently to the nanopore opening and encircled by a β-cyclodextrin (Scheme 8) [69]. At pH 7.0, the resulting pseudorotaxane was highly stable and no cargo leakage was observed, showing that the ‘nanovalves’ remained closed under these conditions. In contrast, upon decreasing the pH at 5.0, protonation of the benzimidazole induced the disassembly of the interlocked components leading to the liberation of doxorubicin. These results indicated that such MSNPs should be stable in plasma (pH 7.2), while releasing the active drug once inside acidic cellular compartments. To verify this hypothesis, the MSNPs loaded with doxorubicin were incubated with squamous carcinoma KB-31 cell lines. These experiments revealed that MSNPs were efficiently taken up into the endosomal compartment where doxorubicin was released under acidic conditions provoking cell death.
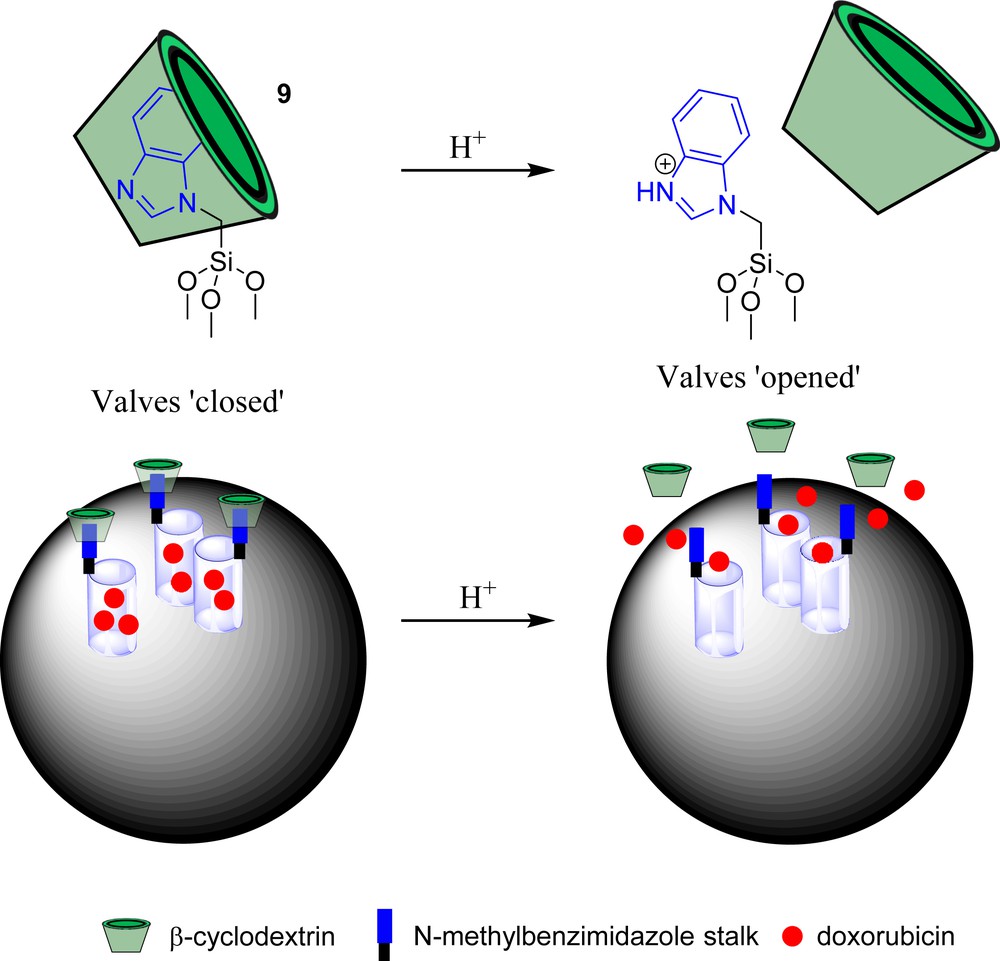
pH-responsive ‘nanovalves’.
Very recently, Zhao et al. studied a range of redox-responsive ‘nanovalves’ comprising a disulfide bond linkage that can be reduced in the presence of intracellular reductive agents such as glutathione (GSH) (Scheme 9) [70–72]. These ‘nanovalves’ were equipped with various targeting ligands allowing the selective recognition of the corresponding membrane receptors overexpressed at the surface of cancer cells. In this approach, following receptor–ligand interactions, the MSNPs are internalized via receptor-mediated endocytosis [73]. Once inside the target cancer cell, the GSH-triggered disulfide cleavage induces the opening of the valves and the subsequent drug release. Based on this principle, Zhao et al. designed MSNPs loaded with doxorubicin and decorated with the pseudorotaxane 10 [71]. This latter included an adamantyl stalk and a lactobionic acid-grafted-β-cyclodextrin serving as an end-capper to prevent drug leakage. The lactobionic acid moiety was employed as a targeting ligand to enter selectively HepG2 liver cancer cells expressing the asialoglycoprotein receptor (ASGP-R) [74]. The anticancer efficacy of such MSNPs was evaluated on tumour-bearing nude mice in vivo. This study demonstrated that doxorubicin was selectively delivered and released within ASPG-R-positive cancer cells thereby inhibiting the tumour growth with minimal side effects.

Redox-responsive ‘nanovalves’.
The same group developed the [2]rotaxane 11 as a redox-responsive ‘nanovalve’ programmed for the selective delivery of doxorubicin inside cancer cells overexpressing the folate receptor (FR) [72]. With this design the folate groups anchored at the surface of MSNPs act as both the stoppers of the rotaxane 11 and targeting ligands. The FR is indeed overexpressed in several cancer types while it is mainly undetectable in most normal tissues [75]. Thus, the FR represents a target of choice to improve the selectivity of cancer chemotherapies [76–78]. Under physiological conditions, a negligible quantity of doxorubicin leaked from the MSNPs, showing a good capping efficiency of rotaxane 11 on the nanoparticle surface. However, in the presence of GSH, doxorubicin was released from MSNPs with a rate depending on the concentration of the reducing agent, indicating that the ‘valves’ opening proceeded as illustrated in Scheme 9. The potential of this targeting strategy was confirmed in vivo during the treatment of a subcutaneous HeLa xenograft implanted in nude mice. In these experiments, the therapeutic efficacy of MSNPs coated with rotaxane 11 was superior to that of the free drug, inhibiting the tumour growth without inducing significant side effects.
Zhao et al. also proposed the use of photothermal-responsive rotaxane-functionalized MSNPs for the controlled release of bioactive compounds in vivo [79]. For this purpose, they attached at the surface of MSNPs the rotaxane 12 in which the cyclodextrin ring was threaded with an azobenzene axle ended by a stopper containing two sulfonic groups, enhancing the aqueous solubility of the whole delivery system (Scheme 10).

Photothermal-responsive ‘nanovalves’.
Upon exposure to visible light or heating, the cis-to-trans isomerization of the azobenzene unit induces the cyclodextrin to shuttle to the trans-azobenzene position, thereby opening the mesopores. On the other hand, irradiation at 365 nm produces the trans-to-cis photoisomerization of the azobenzene that causes the cyclodextrin to move back to the triazole/ethylene glycol position, resulting in the blockage of the pores of the MSNPs. Thus, the control of the movement of the cyclodextrin along the thread allows both drug storage and release. The validity of this concept was confirmed in vivo on zebrafish models with curcumin-loaded MSNPs. Curcumin, a naturally fluorescent polyphenol, was indeed efficiently released when optically transparent zebrafish larvae were treated with either visible light or heat.
6 Concluding remarks
The development of functional rotaxanes for biological applications started to attract interest about fifteen years ago. While this field of research has not reached maturity yet, some stimulating advances have been achieved, particularly in the design of new molecular delivery devices and probes. These systems possess unusual chemical properties due to the presence of mechanical bonds within their structures. Thus, by using the macrocycle of rotaxanes as a molecular shield, some labile chemical moieties can be protected from premature degradation in biological environments. This feature of rotaxane architectures has already demonstrated its usefulness in preventing nucleophilic attack on the cyclobutene core of squaraines and the hydrolysis of peptides or ester bonds in plasma. It was also possible to exploit the mobility of the macrocycle along the thread to develop adaptable host systems allowing the transport of various peptides through cell membranes. Moreover, ‘nanovalves’ and prodrugs, with functionalities based on the controlled disassembly of the mechanically interlocked components, have emerged as innovative drug delivery systems.
Future research directions should focus on the improvement of the biocompatibility of rotaxane-based architectures that usually suffer from poor water solubility. For this purpose, novel versatile synthetic methods allowing the preparation of a wide variety of rotaxanes under aqueous conditions would be extremely useful. Such synthetic advances could lead to the emergence of new families of interlocked systems that could operate in various biological environments, while until now these types of rotaxane-based molecular machines functioned exclusively under abiotic conditions (i.e. artificial muscles and processive enzymes, rotaxane catalysts…). Next generations of functional rotaxanes should also interact more selectively with biological targets in order to compete with non-interlocked molecular systems already developed for similar applications. Within this framework, an important step has been realized very recently by Zhao et al. who developed the first MSNPs coated with ‘nanovalves’ bearing targeting ligands that enabled the selective recognition of malignant cells in vivo.
Although numerous advances still remain to be accomplished to evaluate the real potential of rotaxane-based architectures for biological applications, the functional systems presented in this short review highlight the promise offered by these interlocked molecules. Further developments in this area will certainly permit to determine whatever this class of compounds will be of practical use to explore or manipulate biological systems in the near future.