1 Introduction
As proposed by Jean-Marie Lehn more than two decades ago, supramolecular polymers are formed by low molar mass molecules (monomers) that self-assemble into linear chains held together by reversible and highly directional non-covalent interactions (Fig. 1), such as hydrogen bonds, metal–ligand complexation, π–π interactions, or hostguest interactions [1]. These systems display an obvious structural analogy with polymers, and if the chains are long enough, supramolecular polymers also possess polymer-like rheological or mechanical properties. However, unlike classical polymers, the chains can break and form again at room temperature, which brings additional features, such as self-healing [2], stimuli responsiveness [3] or improved processing [4,5].

Schematic structure of a supramolecular polymer, compared to a fibre from an organogel.
Supramolecular polymers also display a clear analogy with organogelators [6–9], that are indeed formed by self-assembly of low molar mass compounds into anisotropic, usually fibrillar objects. However, the latter systems are in fact often made of intertwined crystalline fibres that have typical cross-sectional dimensions in the 10–100 nm range. This means that organogelators can form much stronger gels than supramolecular polymers; they have for instance been shown to improve the mechanical properties of soft solids such as bitumen [10]. It also means that organogels can rarely heal autonomously after a strong shear and that they are metastable systems that can slowly evolve over time toward a more compact, i.e., more perfect crystal. Therefore, to design a supramolecular polymer with a truly dynamic backbone, one has to incorporate a strong directional interaction while at the same time prevent lateral aggregation of the chains that would lead to irreversible fibre formation.
Over the years, dozens of supramolecular polymers have been reported, and proved to have very diverse structures and properties [11–14]. Most of these systems are synthetic, although there are some very inspiring natural supramolecular polymers such as microtubules and actin filaments, where the dynamic character of the assembly, coupled to an energy dissipation pathway allows intriguing behaviours such as treadmilling [15].
In fact, most synthetic supramolecular polymers known today self-assemble into a fixed, usually predictable, supramolecular structure: the chains are dynamic and their length is polydispersed, but the local structure of the chain is constrained by the structure and the relative positioning of the interacting moieties. In contrast, a particular bisurea-based supramolecular polymer (Fig. 2) was shown to self-assemble in at least two different structures [16]. The increased versatility of this system motivated us to carefully characterize it, and over the last decade, we tried to develop it as a tunable platform of interest in various domains. The aim of the present review is to focus on this particular family of bisurea-based supramolecular polymers. Section 2 summarizes the structural information available, while Section 3 provides an overview of the properties.
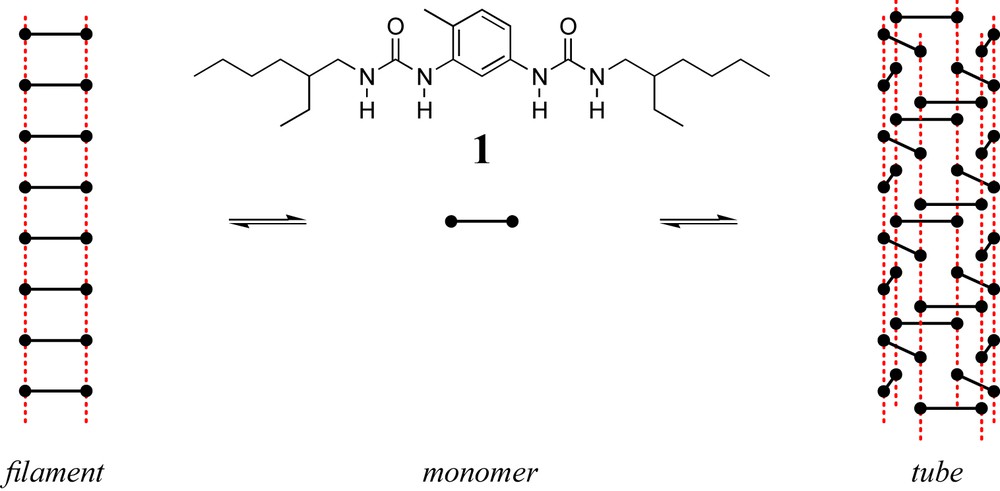
Schematic structure of the filament and tube structures formed by bisurea 1. Hydrogen bonds are represented as red dots.
2 Main supramolecular structures in solution
Bisurea 1 dissolves spontaneously (i.e., heating is not necessary) in non-polar solvents such as dodecane [25], toluene [20] or chloroform [26]. In all cases, FTIR spectroscopy shows the formation of hydrogen bonds between the urea groups and the increased viscosity of the solutions indicates the presence of large assemblies. Whatever the experimental conditions, small angle neutron scattering (SANS) unambiguously proves that the molecules self-assemble into long and rigid rods, but the actual cross-section of the rods depends on the solvent and the temperature [16]. Two supramolecular structures have been identified and have been named “filament” and “tube”, respectively (Table 1).
Influence of solvent and temperature on the respective stability of the filament and tube forms for bisurea 1 (concentrations approximately 10 mM).
solvent | Tube | Filament | Reference |
Dodecane | T < 68 °C | Not observed | [16,17] |
Octane | T < 75 °C | T > 75 °C | [18,19] |
Heptane | T < 73 °C | T > 73 °C | [16,18] |
Cyclohexane | T < 51 °C | T > 51 °C | [16] |
Toluene | T < 43 °C | T > 43 °C | [16,20,21] |
1,3,5-Trimethylbenzene | T < 10 °C | T > 10 °C | [18,22] |
Carbon tetrachloride | Not observed | 22 °C | [16,23,24] |
Chloroform | Not observed | 25 °C | [16] |
2.1 Filament structure
SANS data for bisurea 1 solutions in chloroform or in toluene (at high temperatures) can be quantitatively accounted for by a model for rigid rods [16]. The diameter of the rod cross-section (13 Å) is on the order of the bisurea molecular dimensions, and the measured packing density in the rod indicates an inter-monomer distance of 4 Å. These values are in perfect agreement with the formation of chains of monomers linked by hydrogen bonds and placed such that the cross-section of the filament contains a single monomer. Such scattering data on disordered objects can unfortunately not provide the precise position of the atoms within the filament. Interestingly, molecular mechanics/molecular dynamics (MM/MD) simulations allowed the identification of three distinct hydrogen bonded supramolecular arrangements that are compatible with the SANS data (Fig. 3) [27]. These structures have very similar potential energies, and two of them have actually been identified from the X-ray crystal data of similar bisureas [27]. In all three structures, the monomer conformation is very similar, with dihedral angles between the aromatic and the urea groups close to +140° or −140°. The difference between the three structures actually originates from the frequency of the sign alternation of the dihedral angle along the filament: every monomer, every three monomer or no alternation, for the “straight”, “zigzag” or “helical” structures, respectively. The absence of circular dichroism (CD) activity for bisurea enantiomer 1SS (Fig. 5) in the filament form [28] seems to rule out the “helical” structure, but other than that, experimental data do not allow discrimination between these three potential structures.
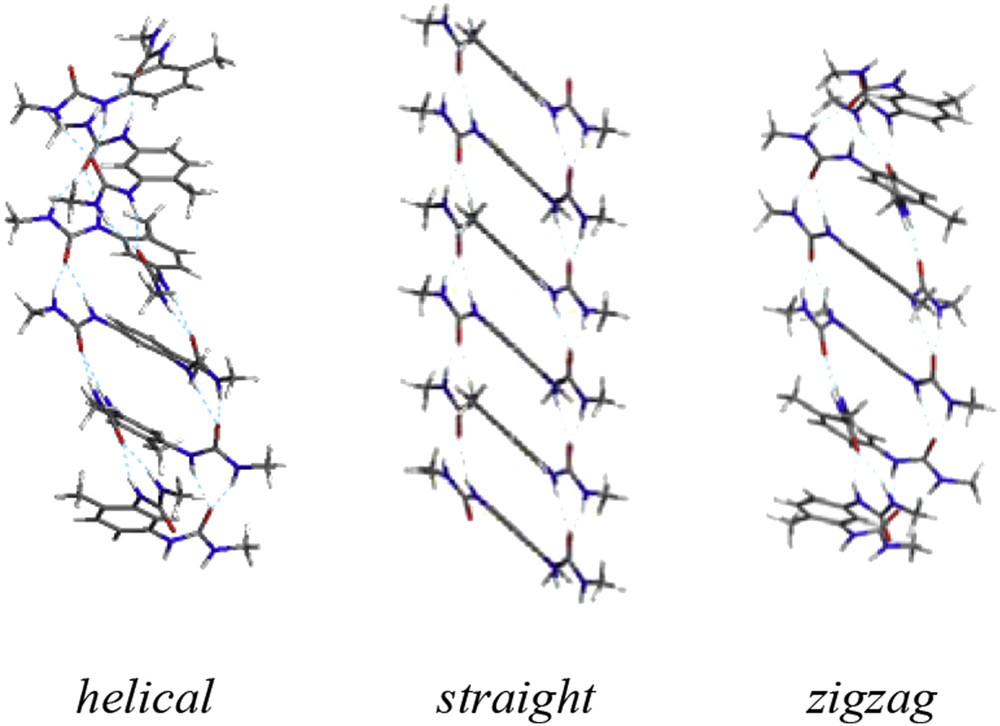
The three possible structures identified by molecular simulation that are compatible with the SANS data of the bisurea 1 filament (the 2-ethylhexyl side-chains have been replaced by methyl groups for clarity) [27].

Structure of the main bisureas studied.
While some uncertainty remains concerning the exact packing within the filaments, their length was characterized in detail by concentration dependent FTIR [26], by light scattering [23] and by isothermal titration calorimetry (ITC) [29]. These data are in quantitative agreement and indicate that the assembly of the filaments displays two levels of cooperativity. The first level of cooperativity is due to the synergistic association of the two urea moieties of a monomer and allows formation of hydrogen bonds at concentrations 50 times lower than for monomers bearing a single urea moiety [24,30]. The second level of cooperativity is revealed by the occurrence of a critical concentration above which the assembly takes place, and by a steep growth of the filaments when the concentration is further increased. This effect is due to both the polarization of the urea function by a first hydrogen bond and the required conformational change of the monomer so that it fits into the filament. Both effects contribute to the higher energetic cost of forming a dimer compared to elongating a preformed oligomer.
The consequence of this cooperative growth is the formation of viscous solutions at millimolar concentrations. The average length of the filaments of course depends strongly on concentration, temperature and the polarity of the solvent, but can reach relatively large values. For instance, at 10 mM and 25 °C in carbon tetrachloride, the number average degree of polymerization of bisurea 1 filaments is about 750 [23] and the relative viscosity of the corresponding solution is 7 [26].
2.2 Tube structure
SANS data for bisurea 1 solutions in dodecane or in toluene (at low temperatures) can also be quantitatively accounted for by a model for rigid rods [16], but the diameter of the rod cross-section (26 Å) is twice as large as that for the filament structure; it actually corresponds to the bisurea maximum molecular dimension. Moreover, the measured packing density in the rod is 2.2 times larger than in the case of the filament, which means that the new objects are two to three times thicker than the previous filaments, i.e., the cross-section of the new objects contains two or three monomers. Other structural information is obtained by FTIR spectroscopy, (all N–H and CO groups are hydrogen bonded) and by dielectric spectroscopy, which shows that no macrodipole is present in the structure (i.e., the urea dipoles are probably placed in an antiparallel orientation and cancel each other) [17]. Moreover, indirect but important structural information can be deduced from the following peculiar solvent effect. The respective stability of the filament structure and the new thicker structure was probed in a range of alkyl-substituted aromatic solvents of similar dielectric properties: a clear correlation between the stability of the thick structure and the molecular width of the solvent molecule was established [22]. For instance, the thick structure is stable up to 43 °C in toluene but only up to 10 °C in 1,3,5-trimethylbenzene. This implies that cavities of about 7 Å diameter are present in the assembled structure, because if the solvent is too bulky to fit in these cavities, the structure is destabilized. Guided by these results, a MM/MD study allowed us to propose a tubular structure built with three molecules in the cross-section (Fig. 4), which is in agreement with this extensive experimental data [17]. For other examples of nanotubes self-assembled from non-macrocyclic monomers, see Refs. [31–34].

Optimized geometry for the bisurea 1 supramolecular tube structure, determined by MM/MD. Reprinted with permission from Ref. [17].
These tubes appear to be extremely long in solvents such as toluene or dodecane, because the ends of the tubes cannot be detected by FTIR spectroscopy. However, the use of ITC together with an adequate mass action law model allows the quantification of the growth of the tubes versus concentration and showing that it is actually even more cooperative than the growth of the filaments [21]. For instance, at 10 mM and 20 °C in toluene, the number average degree of polymerization of bisurea 1 tubes is about 2·104, which corresponds to a curvilinear length of about 4 μm [21]. The huge length, the rigidity and the slow dynamics of these objects are responsible for the appearance of unusual viscoelastic properties that are detailed in Section 3.1.
Moreover, the transition between the filament and the tube structure is very cooperative and can be easily measured by using various techniques. This competition between two supramolecular polymers can actually be used to probe very weak intermolecular effects (see Section 3.2).
2.3 Influence of the monomer structure
The synthesis of bisurea enantiomer 1SS allowed us to further probe the tube structure. Indeed, CD spectroscopy showed that there is a transfer of chirality from the alkyl side chains of the monomer to the urea and aromatic chromophores within the self-assembled tubes; i.e., the 1SS tube has a twisted structure [28]. Moreover, mixing 10% of 1SS with 1 induces a full chiral amplification of the tubes, which means that the tubes formed using bisurea 1 are not straight, but are in fact a racemic mixture of left and right handed twisted tubes.
The straightforward synthetic accessibility of bisureas has allowed us to systematically probe the influence of the structure of the monomer on the properties of the assemblies (Fig. 5). In fact, the main difficulty when designing a new bisurea supramolecular polymer is to ensure that it is soluble in low polarity solvents. Indeed, the urea group is a very polar moiety that tends not only to form strong hydrogen bonds, but also to induce further aggregation in non-polar solvents, as shown by the numerous bisurea-based organogelators described in the literature [35–46]. In particular, when the 2-ethylhexyl groups R2 and R4 of bisurea 1 (Fig. 5) are both replaced by linear alkyls, the solubility in non-polar solvents becomes too low to allow any studies in solution [26]. Therefore, to avoid crystallization, branched substituents should always be part of the design.
A practical way to introduce a branched alkyl without narrowing the synthetic possibilities is to dissymmetrize substituents R2 and R4. Two approaches have been successfully implemented. The first approach consists of a two-step reaction between toluene 2,4-diisocyanate (TDI) and two distinct amines. For instance, bisurea 4 was synthesized by adding 4-butylaniline to an excess of TDI in a non-solvent of the formed mono-urea. A simple filtration allows the removal of the excess TDI, and a second amine (2-ethylhexylamine) can then be reacted [47]. The second approach (bisureas 5n and 6) is a three-step synthesis starting from 2-methyl-5-nitroaniline: formation of the first urea is followed by reduction of the nitro group and finally the second urea is formed [48,49].
Bisureas 3, 4 and 5n proved to be soluble in non-polar solvents and to behave similarly to bisurea 1, although some quantitative differences were noticed. For instance, bisurea 4 forms longer filaments than 1 in chloroform: the five-fold increase in the association constant is probably due to the increased acidity of the aromatic N–H group [47]. It is thus possible to adjust the properties of the supramolecular polymers by fine-tuning the monomer structure.
Another possibility for fine-tuning is to mix two bisureas to form supramolecular copolymers. For instance bisureas 1 and 3 were mixed and the viscosity of the solution was shown to vary smoothly with the monomer composition. Moreover, the transition temperature between the tube and filament forms changes linearly with the monomer composition, indicating a probably statistical distribution of the monomers in the assemblies [50].
While the previous examples illustrate the influence of the outer substituents R2 and R4, it is actually possible to tune the stability of the assemblies more sensitively by changing the substituents on the aromatic spacer. The study of eight bisureas with methyl or ethyl groups placed at various positions (Z1, Z3 and Z5) has allowed the identification of two main effects [51,52]. (1) Alkyl groups in ortho positions to the urea moieties strongly enhance hydrogen bonding because ortho substituents enforce a non-coplanar conformation of the urea and phenyl moieties. This effect is seen both for the filament and for the tube structures. (2) A substituent in position Z3 destabilizes the tube structure compared to the filament, because this position is pointing toward the inside of the tube (Fig. 4), where it causes some steric congestion. These effects are clearly illustrated by the fact that bisurea 7 forms remarkably stable tubes in toluene (down to a concentration of 10−7 M or up to boiling temperature) [51], in comparison to bisurea 1 that form tubes only down to 10−5 M or up to 43 °C in toluene [16], and in comparison to bisurea 8 that does not form tubes, but only filaments under the same conditions [52]. Interestingly, mixing two bisureas with different cores can lead to highly non-linear effects: for instance, in chloroform both 1 and 8 form slightly viscous solutions at 10−2 M and room temperature, because they both self-assemble into filaments. However, a 50/50 mixture of the two solutions yields a viscoelastic gel because of the formation of tubes [52]. This synergistic effect is due to the enhanced preorganization of bisurea 8 and the wider cavities allowed by bisurea 1. Indeed, the presence of bisurea 8 in the mixture is favourable only if its content is low enough, so that the tubular cavities remain large enough to accommodate the solvent molecules.
The preorganization effect demonstrated by alkyl groups in ortho positions to the urea moieties can in fact be further amplified with halogen atoms, because of their electronegativity that is responsible for an enhanced repulsion of the urea oxygen atom. For instance, bisurea 9Cl forms very stable filaments in chloroform (above 4·10−5 M), in comparison to bisurea 8 that forms filaments only above 6·10−4 M under the same conditions [53].
2.4 Influence of the solvent
As for all hydrogen-bonded systems, the polarity of the solvent has a strong influence on the stability of the assemblies. The rheological properties of solutions of bisurea 1 are strongly affected by the addition of polar additives [19], and actually, bisurea 1 does not self-assemble at all in strongly hydrogen bonding solvents such as tetrahydrofuran (THF), ethanol or dimethyl sulfoxide (DMSO). However, it is possible to stabilize the assemblies so that they can better withstand the competition from the solvent. For instance, bisurea 10 was equipped (i) with the same aromatic spacer as bisurea 7 to strengthen self-association, and (ii) with ether substituents to improve its solubility in polar solvents. As a result, bisurea 10 forms viscous solutions in polar liquids such as methylmethacrytate [54], THF, methylethyl ketone or chloroform/DMSO mixtures [55]. It is not clear what the exact structure of the assembly is, but FTIR and SANS unambiguously prove the formation of long rods by hydrogen bonding [55].
In fact, it is possible to enforce assembly even in water by introducing a hydrophobic group in the molecule. Bisureas 11 and 12 were shown to form extremely long rods in water by SANS and cryoTEM [56,57]. These rods are made of approximately 5 molecules in the cross-section that interact both by hydrogen bonds and hydrophobic interactions. Interestingly, calorimetric experiments showed that the assembly is enthalpy opposed and entropy driven, which means that hydrophobic interactions are the main driving force for the assembly. Nevertheless, the comparison with a reference compound unable to form hydrogen bonds proved that the presence of hydrogen bonds is essential to obtain anisotropic rods instead of spherical micelles [56].
In addition to this strong influence of polarity, a more subtle steric effect has been noticed. As mentioned in Section 2.2, solvents with molecular dimensions too large to fit within the tube cavities favour the formation of the filament rather than the tube structure [22]. This effect is responsible for a strong influence of the solvent molecular structure on the viscosity of the solutions. For instance, solutions of bisurea 1 are 200 times more viscous in p-xylene than in o-xylene [58].
3 Main properties and main systems derived
3.1 Rheology of dilute and semi-dilute solutions
The obvious consequence of the formation of long objects is that above a certain concentration, these objects overlap and are responsible for a strong increase in the viscosity of the solution. Under semi-dilute conditions, the self-assembled tubes actually get entangled and form visco-elastic solutions, which means that their behaviour is mainly elastic at high frequency and viscous at low frequency. Indeed, the self-assembled tubes present in the sample are robust enough to withstand the mechanical solicitation at high frequencies, but given enough time (at frequencies on the order of 0.1–1 rad s−1), the tubes can break and diffuse to relax the applied stress. These soft gels can be of interest in the context of various applications in particular in the fields of cosmetics [59–62] and hydrocarbons [63–65], and their rheological properties have been studied in detail in the linear domain [17,25], but also in the non-linear domain where shear-banding has been observed [66], by large amplitude oscillatory shear [67], and by microrheology [68].
At very low concentrations (typically about 0.5 g/L) the supramolecular tubes are not entangled, but are still long enough to show typical polymer-like properties such as hydrodynamic drag reduction [19,69], with the added advantage that, unlike high molar mass polymers, they do not degrade under shear.
Because of the reversible nature of the backbone of these polymer-like systems, several stimuli can be used to tune their properties, such as temperature [17], concentration [25], or the addition of a co-monomer [50], a co-solvent [19] or a chain-stopper [23]. Chain stoppers are designed to interact specifically with the extremities of the supramolecular polymer and therefore reduce the length of the assemblies and thus the viscosity of the solutions. Various chain stoppers (Fig. 6) have been derived from the bisurea monomer structure by reducing its hydrogen bond donor ability by simple alkylation of the urea group (S1, S2, S6). Another approach is to replace the urea by a thiourea group, which is a stronger hydrogen bond donor and, at the same time, a weaker hydrogen bond acceptor than the urea group [70]. The net effect is that self-assembly is less optimal, and bisthiourea S3 revealed itself as a poor supramolecular polymer, but an efficient chain stopper [71]. Actually, simple anions (Fig. 6) have been identified as extremely efficient chain stoppers, leading to a decrease in the viscosity of bisurea 1 solutions by a factor of 10 for a molar fraction of the stopper of only 10−5 [72]. These chain stoppers can obviously be used to reduce the viscosity of the solutions, but they are also extremely useful as a characterization tool, because they allow control of the chain length of the assemblies independently of the monomer concentration [23,73–76]. Finally, chain stoppers can also be used to functionalize the extremities of the supramolecular chains, for instance with polystyrene chains (S6, Fig. 6) [77].
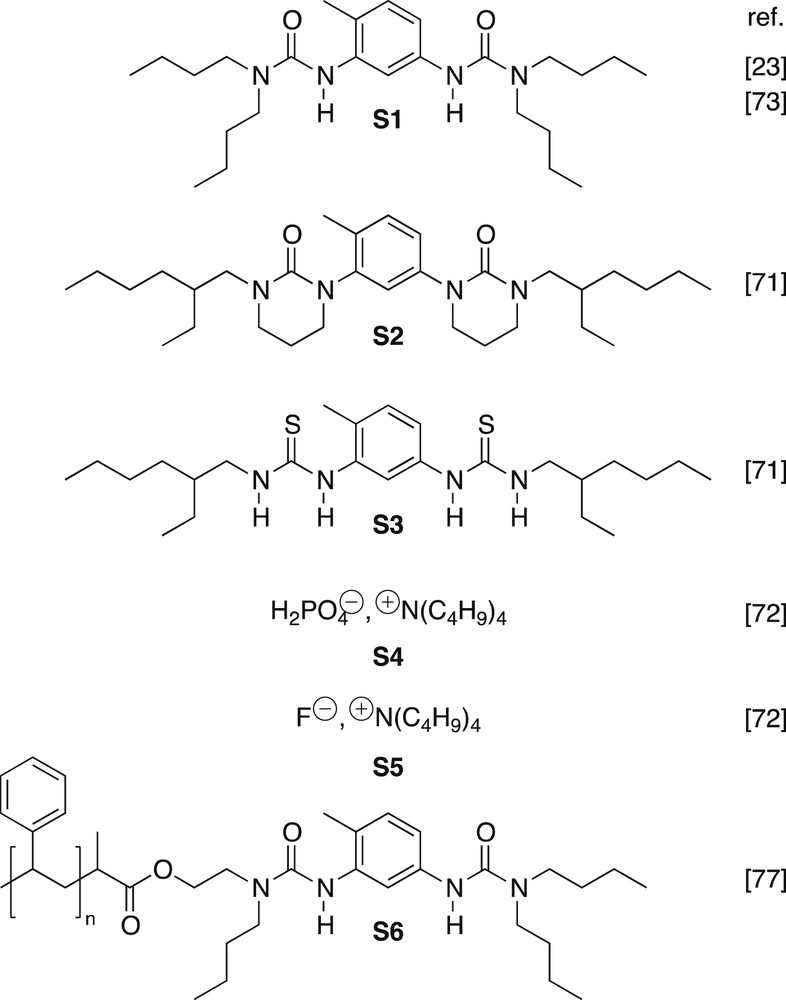
Structure of chain stoppers.
3.2 Supramolecular balance
While the transition from filament to tube is of interest to modulate the macroscopic rheological properties of a solution, it can also be exploited at the molecular level to probe weak intermolecular interactions. Indeed, because of the much tighter packing of the monomers in the case of the tube structure, intermolecular interactions within the tube can be expected to be significantly different from those within the filament. Comparison of the transition temperatures of two similar bisureas can therefore yield quantitative information on the interaction that is present in one system and not (or less) present in the other [48]. Because of its high level of cooperativity, the transition between tubes and filaments is very sharp and is detected by an endothermic peak in a differential scanning calorimetry (DSC) experiment performed with a dilute solution [21]. A difference in the transition temperature of 1 °C corresponds to a free energy difference of about 10 J/mol [48], which makes this supramolecular balance more sensitive by two orders of magnitude than the existing molecular balances that are based on NMR data [78]. As a proof-of-concept of the use of the bisurea platform as a supramolecular balance, we probed the strength of van der Waals interactions between alkyl or alkene groups in toluene solution. Replacing the terminal ethyl groups of 1 by terminal vinyl groups (2) destabilizes the tube structure (or stabilizes the filament structure) by 110 ± 5 J/mol. A plausible interpretation is that a steric repulsion between vinyl and alkyl groups is at the origin of the observed destabilization in the tube form. Alternatively, the destabilization of the tube form may arise from a favourable solvation of the vinyl group by toluene in the filament form. In order to discriminate between such effects, we need to probe the tube/filament transition of a full set of structurally-related bisurea monomers in a range of solvents of differing polarities and polarizabilities.
This supramolecular balance concept was also successfully applied in the case of mixtures of enantiomers, to quantify the energetics and statistics of defects that occur within self-assembled, helical, rod-like objects [79]. As previously mentioned, bisurea 1 exists as a mixture of right and left-handed helical tubes, and doping 1 with 10% of 1SS (or 1RR) is enough to get helices with a single handedness [28]. This chirality amplification phenomenon is known as the majority-rules effect and has been observed for several types of helical supramolecular polymers [80]. The efficiency of chirality amplification is related (i) to the ability of a given monomer to be incorporated in a helix of its non-preferred screw sense (mismatch) and (ii) to the occurrence of reversals in the helical screw sense along the chain. Both situations constitute defects that directly affect the stability of the assemblies. By means of the supramolecular balance concept, we have used calorimetric experiments to quantify the energetics and statistics associated with these defects. A simultaneous fit of both helicity (CD experiments) and stability (DSC measurements) data yields the following values for the helix reversal penalty HRP = 19.5 kJ/mol and the mismatch penalty MMP = 0.05 kJ/mol. These values imply that helix reversals are very rare (but can still occur a few times along the tubes), and that nearly half of the monomers are mismatched [79]. This is actually a unique approach to study the energetics associated with chiral amplification phenomena by means of experimental techniques other than chiroptical spectroscopies.
Finally, the supramolecular balance concept was also applied to probe confinement effects for solvent mixtures within the self-assembled bisurea nanotubes [18]. Indeed, a non-linear dependence of the filament–tube transition temperature of 1 on the bulk solvent composition has been demonstrated and can be quantitatively accounted for by a model taking into account the interaction free energy between the neighbouring solvent molecules that are placed in a single file configuration, in contrast to the other solvent molecules, that are in the bulk mixture and that are less ordered.
3.3 Assemblies on surfaces
Scanning tunneling microscopy (STM) has allowed us to visualize monolayers formed by bisurea 1 on a Au(111) surface (Fig. 7) [81] and to perform tunnelling spectroscopy measurements [83]. Intermolecular hydrogen bonding is the main interaction governing the assembly, but van der Waals interactions between the branched alkyl groups also play a significant role [84]. Remarkably, large domains were found to cross monoatomic steps on the substrate without perturbation of their order (Fig. 7, bottom), which shows that, in this case, the coupling to the substrate is overruled by intermolecular interactions [82]. More generally, the relative importance of the molecular structure (branching) and the molecule–substrate interaction was investigated in detail on carbon substrates (graphene and graphite) [85].
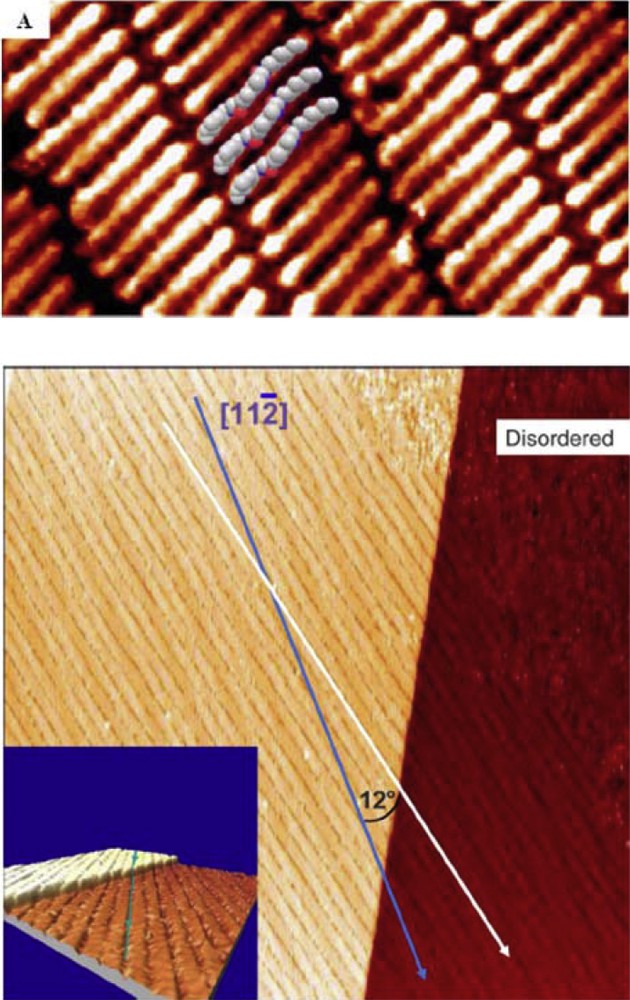
Top: High resolution STM image (5*10 nm2) with insets of a space filling model of bisurea 1 on Au(111). Bottom: Topographic image (100*100 nm2) of a large supramolecular layer that crosses a monoatomic step (about 0.24 nm) of the underlying gold substrate. Reprinted with permission from Refs. [81,82].
3.4 Polymer modification and assemblies in bulk
We also investigated the use of bisurea stickers to modify the properties of polymers. Various complementary synthetic approaches are available, such as polymer modification, chain coupling or polymerization from a functional initiator (Fig. 8). Several polymer backbones (polysiloxane [86], polyisobutene [87], poly(ethyleneglycol) [57], polystyrene [88], polyacrylates [89]) and several stickers (mono-, bis-, tri-ureas) were combined according to several macromolecular architectures (Fig. 9). It appears that the properties of the final system depend strongly on the chosen architecture (in particular on the number of stickers per chain) and on the dynamics of the stickers, i.e., whether the assembled stickers are in a dynamic exchange or are frozen at the temperature of the experiment.

Strategies developed to introduce a bisurea sticker into a macromolecular architecture.

Macromolecular architectures prepared with bisurea stickers.
Light and neutron scattering data show that polymers with a single sticker in the middle of the chain (Fig. 9a) form bottle-brush structures [87–90], the size of which depends sensitively on the competition between the self-association of the sticker and the steric hindrance of the polymer arms [88,90]. In the case of a trisurea sticker, a backbone length larger than hundreds of nanometres can be obtained [90]. In the absence of solvent, the rheological properties of these systems are strongly affected by the stickers, even though no cross-linking of the chains can occur [91–93]. Of particular note are the exceptional adhesive properties (both on steel and on silicone surfaces) displayed by a polyisobutene functionalized by a bisurea sticker, that apparently allows an unusually strong dissipation upon deformation of the sample (Fig. 10) [91].
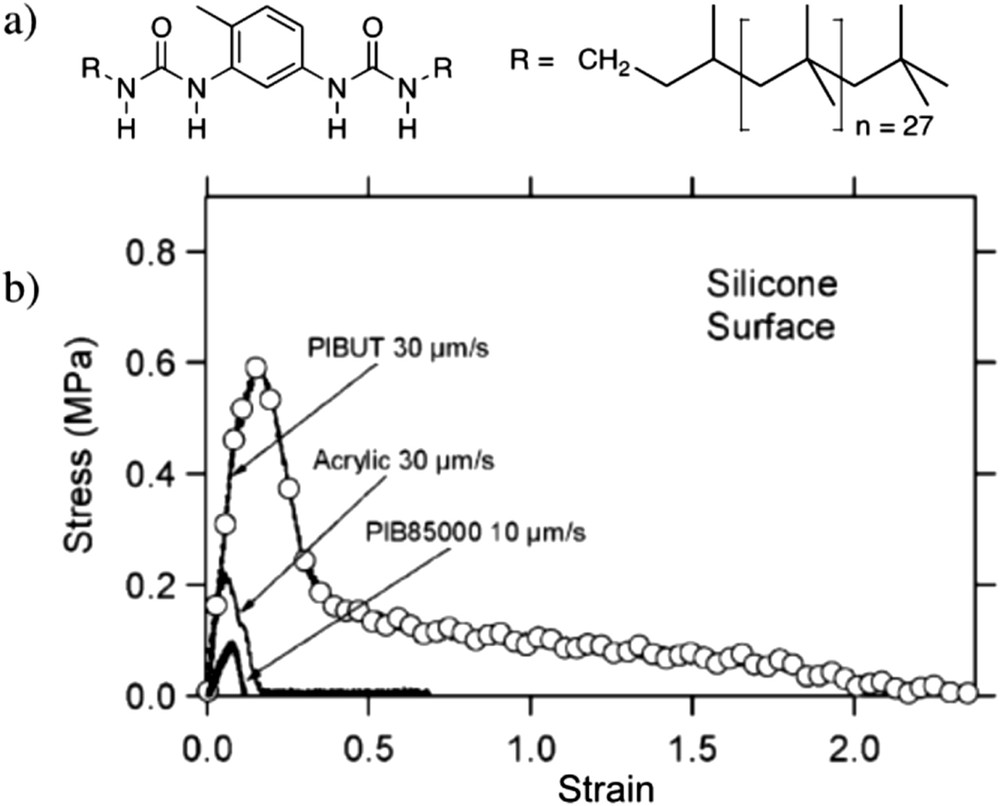
Bisurea functionalized polyisobutene: structure (a) and adhesion tests (probe tack experiment) on a polydimethylsiloxane (PDMS) surface, compared to reference materials (acrylic adhesive and non-functional polyisobutene of molar mass 85 kg/mol) (b).
When two or more stickers are present on the chains, gel formation can be favoured in solution [57], and interesting thermoplastic elastomers can be obtained in bulk [86].
4 Outlook
The straightforward synthetic accessibility of the bisurea synthon has made it possible to explore its rich self-assembly behaviour. Under suitable conditions, the assembly of low molar mass bisureas can lead to potentially interesting monolayers or viscoelastic solutions. Moreover, polymers with improved rheological or adhesive properties can be designed by grafting bisurea stickers. However, the most original behaviour of these systems comes from the fact that two supramolecular structures are in competition. This has allowed us to develop bisureas as a versatile platform to probe weak intermolecular interactions. Our current goal is to further develop this platform in the fields of supramolecular catalysis [94] and stimuli responsive gels.
Acknowledgements
The numerous students and colleagues who helped us in these studies and whose names appear in the references are gratefully acknowledged.
1 Dedicated to Professor Jean-Pierre Vairon on the occasion of his 78th birthday.