1 Introduction
Copper is a very useful metal, because it finds many daily applications including, for example, manufacturing of electric cables and thermal pipes, coating materials for buildings, chemical catalysis and wood pulp production [1]. Copper is also an essential trace element that is vital to the health of living organisms (humans, plants and animals). However, at elevated concentration, it becomes detrimental to these organisms [2]. Yet, copper is known to be one of the most toxic heavy metals and one of the most widespread contaminants in the environment [3–7]. Several conventional methods are traditionally used to remove heavy metals from wastewater. However, the application of such technologies is restricted because of technical or economical limitations.
For a few years, efforts have been made for the improvement and the setting-up of new more effective, less expensive and especially ecological processes. Natural adsorbents such as clays and silica have been largely used for the elimination of pollutants [8,9].
In recent years, considerable attention has been focused on removal of heavy metal ions (or other pollutants) from aqueous solution using lignocellulosic materials (LCMs) [10–13] for several reasons: LCMs are naturally available in abundance, inexpensive, non-toxic and able to adsorb various pollutants at low concentration [12]. Moreover some of these materials can be regenerated and reused [14,15]. LCMs bear various functional groups such as hydroxyl, carboxyl, amine, amide, thiol, etc. that can easily form complexes or chelates with heavy metal ions [11–14].
The aim of this work was to study the biosorption of copper(II) from aqueous solutions using two LCMs: maize spatha (MS) and newspaper scraps (NS). MS are agricultural residues, defined as large foliaceous envelopes that protect the corncob. Paper is a material which was first manufactured from cellulose fibers contained in wood or other woody plants. Paper scraps are agro-industrial residues remaining after the cutting of coarse papers. The influence of several parameters (contact time, biosorbent dosage, shaking speed, pH of solution, initial concentration of the pollutant, and ionic strength) on the biosorption of Cu(II) in batch mode was studied. The involved process was described by using equilibrium and kinetic data of biosorption. The possibility to reuse the materials was also examined.
2 Materials and methods
2.1 Sample1
Newspaper scraps were collected from the printing workshops of the University of Dschang (Cameroon). Maize spatha were obtained from a local agricultural unit. They were sundried for 3 days under open atmosphere, cut up and crushed as finely as possible using a domestic mixer. The obtained powders were sieved into different fraction sizes (ranging from 0 to 200, 200 to 250 and 250–500 μm). Experiments were carried out with the smallest fraction size (0–200 μm) which exhibited the best results towards the adsorption of Cu(II) ions.
All the chemical reagents were purchased and used as received: CuSO4.5H2O from Acros organics; NaCl, MgCl2, CaCl2, SnCl2, FeCl3, NH4OH, and NaOH from Fischer; CH3OH and H2SO4 from BDH; KCl, NaNO3, Na3PO4 and Na2SO4 from Prolabo; HNO3 from Riedel-de-Häen. All the solutions were prepared with distilled water.
2.2 Pretreatment of biomass
Pretreatment with methanol was performed according to the procedure used by Nanseu-Njiki et al. [14]. Pretreatment with nitric acid was carried out as follows: into a bottle containing 5 g of biosorbent, a volume of 50 mL of 2 M HNO3 was introduced. The mixture was kept under agitation at a speed of 200 rpm for 2 h, and then filtered. The obtained product was washed with distilled water to dilute and eliminate excess of nitric acid. Afterwards, the mixture was immersed in 0.1 M NaOH for 1 h in order to eliminate the rest of HNO3 and to activate the functional groups found on the surface of biosorbents. The obtained products (NSHNO3 and MSHNO3) were once more washed with distilled water to eliminate the rest of NaOH. They were sundried for two days, and then kept in an oven at 105 °C for 2 h.
2.3 Batch biosorption studies
The biosorption was carried out in the batch mode. Into a 100 mL flask, a given mass of biosorbent and 10 mL of Cu(II) solution at a given concentration were introduced. The pH was adjusted to desired values with solutions of NaOH or HCl. A series of flasks were prepared by varying the different parameters to be investigated. The flasks were placed in a mechanical platform shaker (Edmund Bühler, GmbH) and stirred for 40 min at a speed of 150 rpm.
The performance of biomaterials for the removal of Cu2+ ions from aqueous solutions was quantitatively evaluated using the amount of Cu2+sorbed per unit mass of biosorbent (mol/g):
(1) |
The percent biosorption of metal ions (R, %) was calculated as follows:
(2) |
Cu(II) concentrations were spectrophotometrically measured: to 5 mL of Cu(II) solution, 2.5 mL of 10 M ammonia was added and the mixture was allowed to stand for about 5 min. The absorbance of the corresponding blue solution formed was measured at 610 nm using a Jenway UV–Vis spectrophotometer. For the determination of residual Cu(II) concentrations in filtrates where the concentration of this analyte was below 10−4 M, an organoclay modified electrode prepared by our group according to a previously published procedure [16] was used. Briefly, a carbon paste electrode chemically modified by a smectite clay bearing amine was used as the working electrode in a three electrode cell configuration comprising a tungsten wire counter electrode and a saturated calomel electrode (SCE) used as the reference electrode. Square wave anodic stripping voltammograms were recorded using a Palmsens potentiostat. The electrochemical analyzing procedure involved two successive steps: an open-circuit preconcentration of Cu(II) followed by voltammetric detection in 0.2 M HCl solution. The first step was achieved by dipping the working electrode in the filtrate containing Cu2+ ions, under stirring. After 4 min accumulation, the electrode was promptly removed, rapidly rinsed with water and then transferred into the electrochemical cell containing the detection solution. The previously accumulated species were then analyzed by square wave voltammetry after 60 s electrolysis at −0.8 V. The concentration of Cu(II) was finally determined using a calibration curve previously established (see Fig. S1 and S2, supporting information).
2.4 Desorption
Each LCM (20 mg) was contacted with 10 mL of a 5 × 10−3 mol/L Cu(II) solution. Upon agitation for a given period of time, the LCM was collected by filtration and washed three times with distilled water, to remove residual Cu(II) on the surface. It was then transferred into 10 mL desorbent solutions (0.001 M–0.1 M HCl). The mixtures were agitated for 1 h, then the filtrates were analyzed to determine the concentration of Cu(II) after desorption. The desorption ratio was calculated from the amount of metal ion uptake by the LCM and the percentage of desorption was obtained using Eq. (3):
(3) |
3 Results and discussions
3.1 Effect of chemical pretreatment on the biosorption of Cu(II)
Some researchers have reported that the biosorption capacity of LCMs can be improved upon chemical treatment [11]. Thus, MS and NS were pretreated using nitric acid and MeOH. Fig. 1 shows the amount of Cu2+ adsorbed by NS and MS, before and after pretreatment.
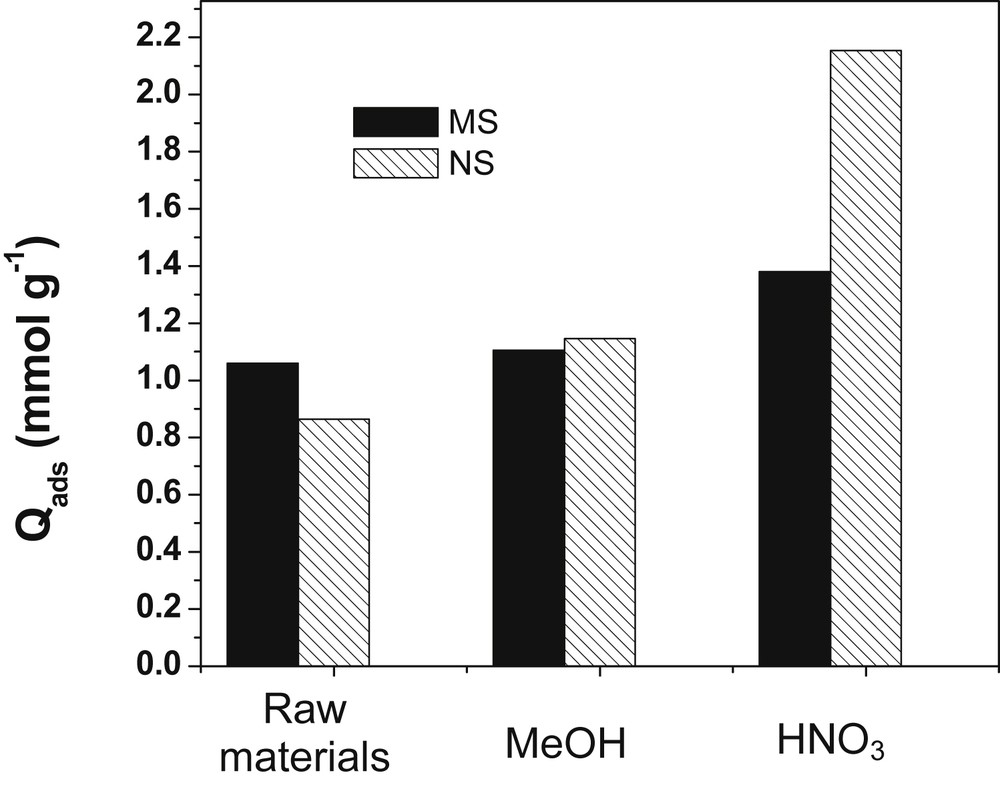
Effect of pretreatment of biomass on the biosorption of Cu(II) ions. Experimental conditions: [Cu2+]: 5 × 10−3 mol/L; biosorbent dosage: 2 g/L; contact time: 40 min; 150 rpm.
Regarding the raw materials, raw MS have a more important sorption capacity (1.06 mmol/g) than raw NS (0.865 mmol/g). Meanwhile, NS treated with methanol (NSMeOH) or with nitric acid (NSHNO3) present better sorption capacities (1.255 and 2.154 mmol/g, respectively) than MS treated with methanol (MSMeOH) and the nitric acid MSHNO3 (1.125 and 1.380 mmol/g, respectively). These observations suggest that raw NS may contain more extractables than pristine MS. These extractables may congest NS pores, thereby diminishing their sorption capacity. Once NS are treated, their sorption capacity increases significantly compared to MS. Thus, the material containing more extractables has high sorption capacity upon chemical pretreatment that makes its pores more accessible. In this case, the diffusion of Cu(II) ions to the active sites on NS and MS is easy. Therefore, the study of other parameters was carried out with NSHNO3 and MSHNO3.
3.2 Effect of the biosorbent dosage on Cu(II) biosorption
For a given volume and initial concentration of Cu(II) ions (precisely 10 mL of 5 × 10−3 M Cu(II)), the biosorbent dosage capacity defined by the biosorbent dosage on Cu(II) removal was examined by using the dosage in the range from 0.0 to 65 mg/10 mL as shown in Fig. 2.
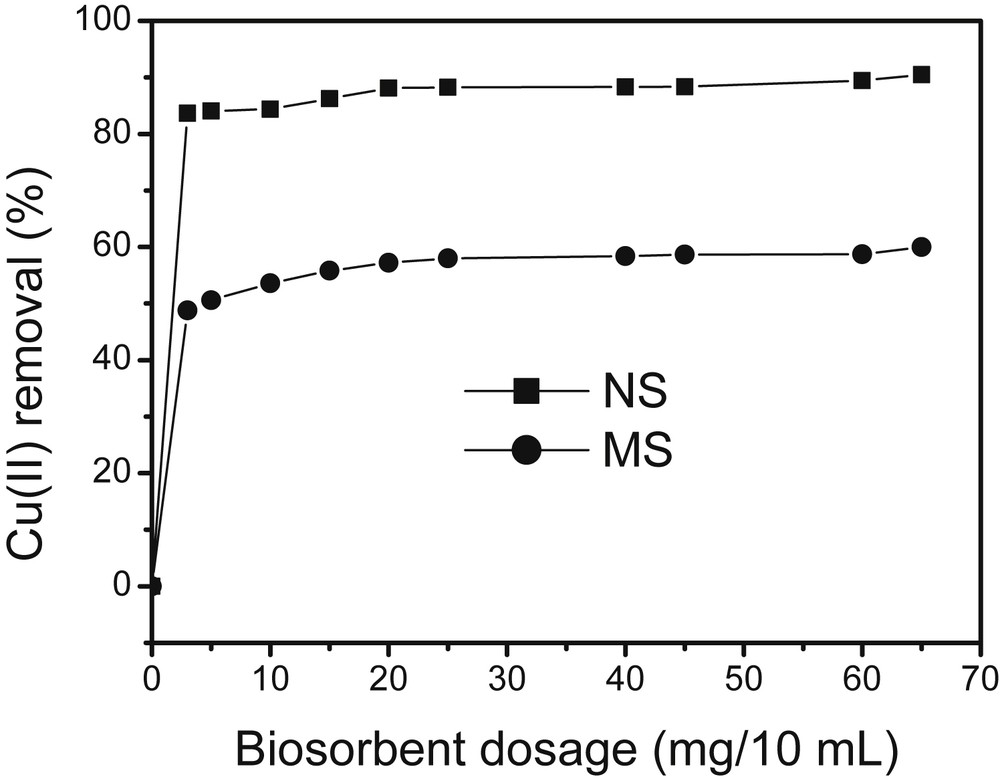
Effect of biosorbent dosage on the biosorption of Cu(II) ions. Experimental conditions: [Cu2+]: 5 × 10−3 mol/L; contact time: 40 min; 150 rpm. Volume of sorbate: 10 mL.
It was observed that an increase in the adsorbent dose from 0.25 to 2 g/L resulted in an increase in Cu(II) ion biosorption from 48 to 84% for MSHNO3, and from 84 to 90% for NSHNO3. A further increase in the adsorbent dosage (≥2 g/L) for both materials did not cause significant improvement in biosorption as a consequence of an equilibrium established between Cu(II) ions bound to the sorbents and those remaining in solution. Such a behavior was expected since an increase in adsorbent mass implies the availability of more adsorption sites since copper concentration is kept constant. Globally, one observes an increase in the sorption percentage of Cu(II) ions with the increase in the mass of biosorbents. Several authors obtained similar results during the biosorption of the same analyte on various types of lignocellulosic materials [17,18]. The results obtained indicated that a dosage of 2 g/L of biosorbent could be sufficient for the optimum removal of Cu(II) ions, a value which was used for further experiments.
3.3 Effect of shaking speed
The shaking speed is a parameter that can influence the kinetics of adsorption. Thus, keeping the biosorbent dosage of NS and MS at 2 g/L and the concentration of Cu(II) ions at 5 × 10−3 mol/L, the shaking speed was varied from 50 to 300 rpm (Fig. 3).
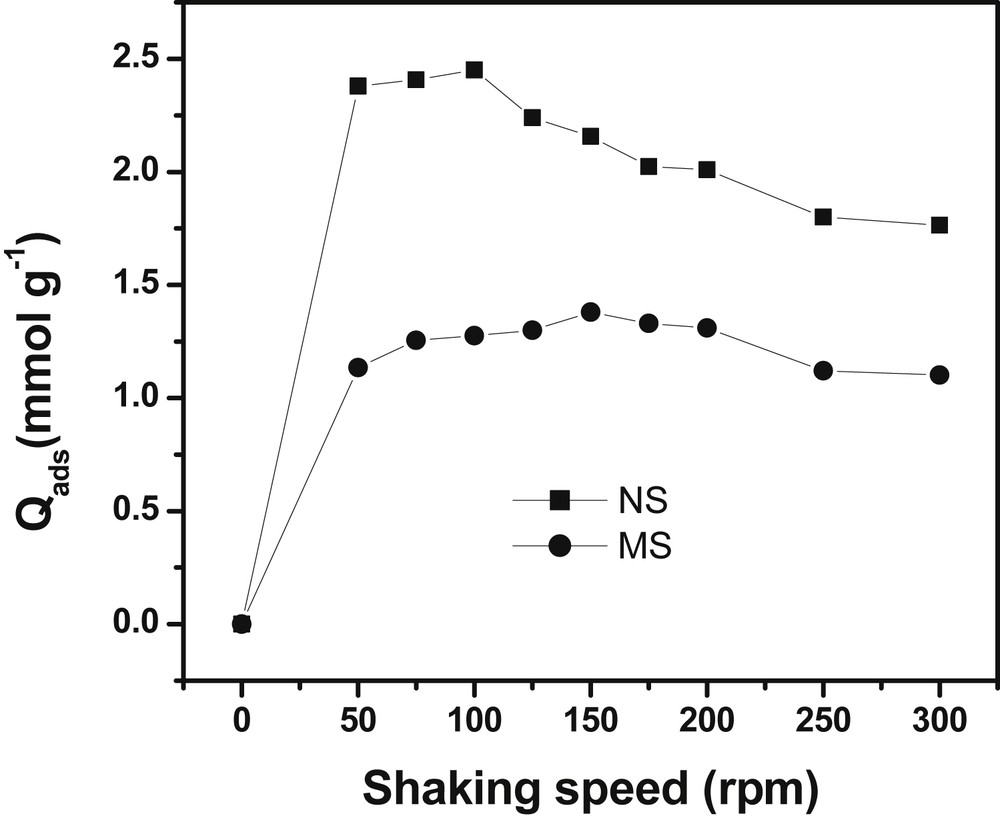
Effect of shaking speed on the biosorption of Cu(II) ions. Experimental conditions: [Cu2+]: 5 × 10−3 mol/L, Biosorbent dosage: 2 g/L, contact time: 40 min (NSHNO3) and 20 min (MSHNO3).
The results in Fig. 3 show that increasing agitation speed up to 100 rpm for NSHNO3 and 150 rpm for MSHNO3 increases the sorption capacity of the cupric ions. When the mixture is agitated, the solid particles move quickly in the solution, thus increasing the concentration of metal in the vicinity of their surface, probably towards a value close to the concentration within solution [19]. One can notice that the sorption capacity of copper (II) by the NSHNO3 decreases more significantly from 2.45 mmol/g to 1.765 mmol/g beyond 100 rpm. This feature can be explained by a release of Cu(II) ions by the NSHNO3 material at speed higher than 100 rpm. As a conclusion, one may consider that there is an optimal shaking speed, sufficient to support the contact between biosorbent particles and Cu(II) ions, and sufficiently weak not to break the forces of sorption [20]. It is noted that at low agitation speed, the retention of Cu(II) ions is the highest.
3.4 Effect of the ionic strength
The choice of NaCl as the salt was justified by the fact that most effluents contain in major part chloride and sodium ions. The concentration of NaCl was thus varied in order to evaluate the effect of ionic strength on the biosorption of Cu(II). The results obtained are represented in Fig. 4.

Effect of ionic strength on the biosorption of Cu(II) ions. Experimental conditions: [Cu2+]: 5 × 10−3 mol/L; m = 20 mg; granulometry: 0–200 μm, shaking speed: 150 rpm (MSHNO3) and 100 rpm (NSHNO3), V = 10 mL, contact time: 40 min (NSHNO3) and 20 min (MSHNO3).
It can be seen that an increase in salinity decreases the sorption capacity of Cu(II) ions for both biosorbents. However, the sorption of Cu(II) decreases significantly when one passes from a solution free from NaCl to a 0.01 M. This sorption capacity decreases more significantly when the concentration of salt is varied from 0.01 to 0.1 M. This confirms that there is indeed a competition between the Cu2+ ions and Na+ ions for the occupation of the same sorption sites, the sorption process proceeds thus by a cation exchange mechanism [21]. But, the presence of Na+ ions in the studied concentration range does not influence notably the elimination of the Cu2+ ions. As for chloride ions, previous studies related to the sorption of Cu(II) ions showed that the type of anion present in the effluent to be treated affects very little the sorption capacity [22].
3.5 Effect of interfering ions
Effluents in general contain high concentrations of various ions, and it is well known that the sorption of ionic species on a solid can be influenced by the presence of other ions in solution. The study of the influence of several species was thus undertaken on Cu(II) biosorption by NSHNO3 and MSHNO3. The investigated ions include Na+, K+, Mg2+, Ca2+, Sn2+ and Fe3+ as cations; and , and as anions. These species were introduced in turn in the solution containing Cu(II) ions, and their concentration was fixed at 0.01 M, a value sufficient to induce interferences with Cu(II) as revealed by the results obtained in the previous section during the analysis of the effect of ionic strength. The results obtained are shown in Fig. 5 for cations while the data relative to anions are provided as Supporting information (Fig. S3). As the main results, the presence of cations decreased the biosorption of Cu(II) by both biosorbents.
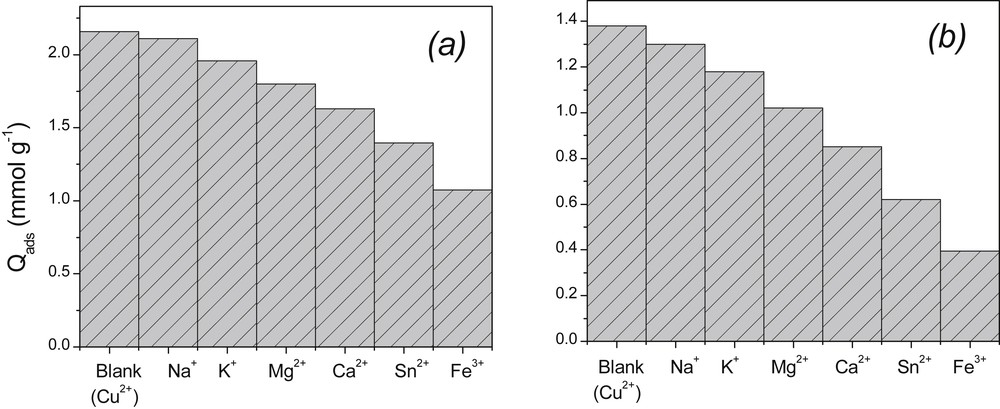
Effect of interfering ions on the biosorption of Cu(II) ions. Experimental conditions: [Cu2+] = 5 × 10−3 mol/L; m = 20 mg; shaking speed: 150 rpm (MSHNO3) and 100 rpm (NSHNO3), V = 10 mL; contact time: 40 min (NSHNO3) and 20 min (MSHNO3).
The inhibition of the uptake of Cu(II) follows the order Fe3+ > Sn2+ > Ca2+ > Mg2+ > K+ > Na+. The cations with a high charge density greatly compete for the occupation of the same sorption sites. A similar observation was made for anions, as the influence of ions towards the biosorption of Cu(II) was more pronounced compared with and (See Fig. S3, supporting information).
3.6 Effect of pH solution on Cu(II) biosorption
The pH is a remarkable parameter that can influence biosorption of metal ions. Yet, the competitive ability of H+ species with other cations to the active sites on a sorbent surface is affected by the pH of the solution. The effect of solution pH on Cu(II) biosorption by NSHNO3 and MSHNO3 was investigated by using 2 g of each biosorbent per liter of 5 × 10−3 M Cu(II), and the results are shown in Fig. 6. For both materials, the biosorption of Cu(II) was relatively low for a strong acidic medium (pH 2), increasing slightly from pH 2 to pH 5. Between pH 5 and pH 6, the uptake of Cu(II) by biosorbent materials increased sharply, and then remained almost constant up to pH 9.
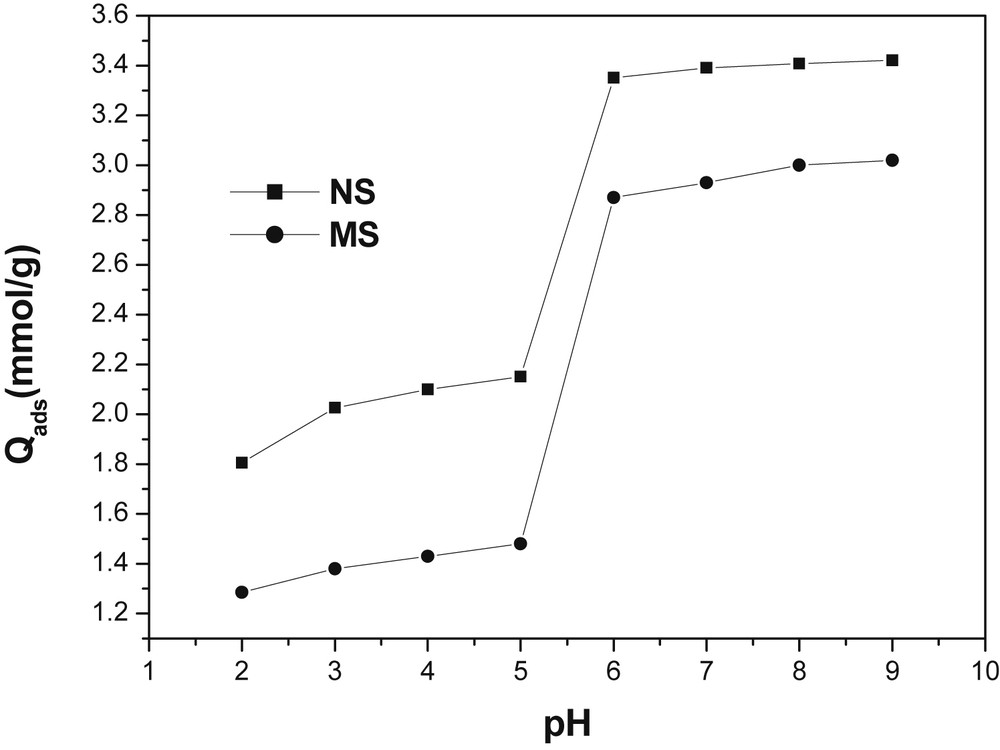
Effect of pH on the biosorption of Cu(II) ions. Experimental conditions: [Cu2+]: 5 × 10−3 mol/L; m = 20 mg; granulometry: 0–200 μm; shaking speed: 150 rpm (MSHNO3) and 100 rpm (NSHNO3), V = 10 mL, contact time: 40 min (NSHNO3) and 20 min (MSHNO3).
This could be explained by the fact that with low values of the initial pH, the surface of the sorbent is surrounded by H+ ions that decrease the interactions between the cupric ions and the active sites of the sorbent. Thus, the reduction in the rate of sorption at low pH values can be due to the high concentration and the great mobility of the H+ ions which are preferentially fixed by the sorbents [23]. With the increase in the pH, the total surface of the sorbent becomes negatively charged and consequently the adsorption of Cu(II) is favored. The results of the sorption of Cu(II) ions on NSHNO3 and MSHNO3 materials show that optimal elimination is obtained in the basic range of pH.
3.7 Sorption isotherms
In order to better describe the uptake of Cu(II) by NSHNO3 and MSHNO3 materials, three isotherm models (Langmuir, Freundlich and Temkin) were applied. In fact, interactions between the sorbate and adsorbents are indicated by sorption isotherms, and the initial metal concentration provides an important driving force to overcome all mass transfer resistances between the aqueous and solid phases. Isotherms are often exploited for the determination of the maximum sorption capacities of the pollutants and for the identification of the type of sorption. The nonlinear and linear formulae of Langmuir [24] are respectively represented by the following equations:
(4) |
(5) |
(6) |
(7) |
The Temkin isotherm is generally presented by the following equation:
(8) |
Qmax (mmol g−1) and KT (L mmol−1) are Temkin constants, R is the ideal gas constant (8.31 J mol−1 K−1), Ce is concentration of ions with the metal equilibrium (mmol L−1) and T is absolute temperature (K) [26,27].
For NSHNO3 and MSHNO3 materials, the Langmuir, Freundlich and Temkin isotherm models are illustrated in Fig. 7 and Fig. 8, while the corresponding constants are gathered in Table 1.
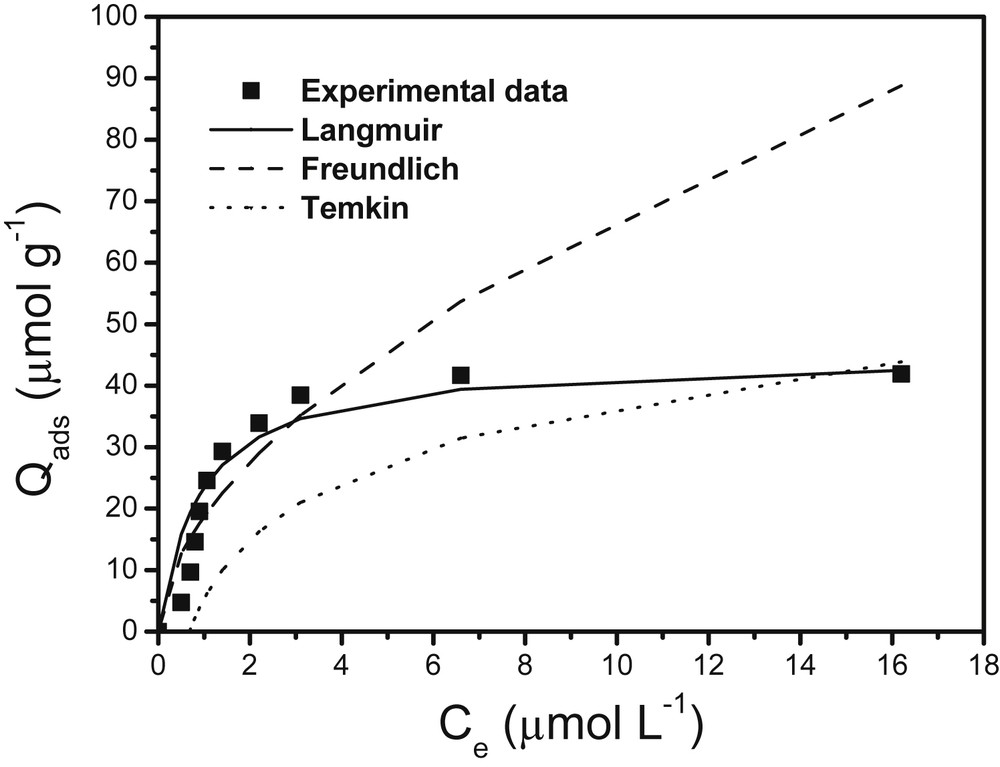
Variation of the concentration of Cu(II) ions sorbed at the equilibrium in solution on NSHNO3. Simulation models of Langmuir, Freundlich et Temkin. Experimental conditions: m = 20 mg; granulometry: 0–200 μm; contact time: 40 min; shaking speed: 100 rpm; V = 10 mL.
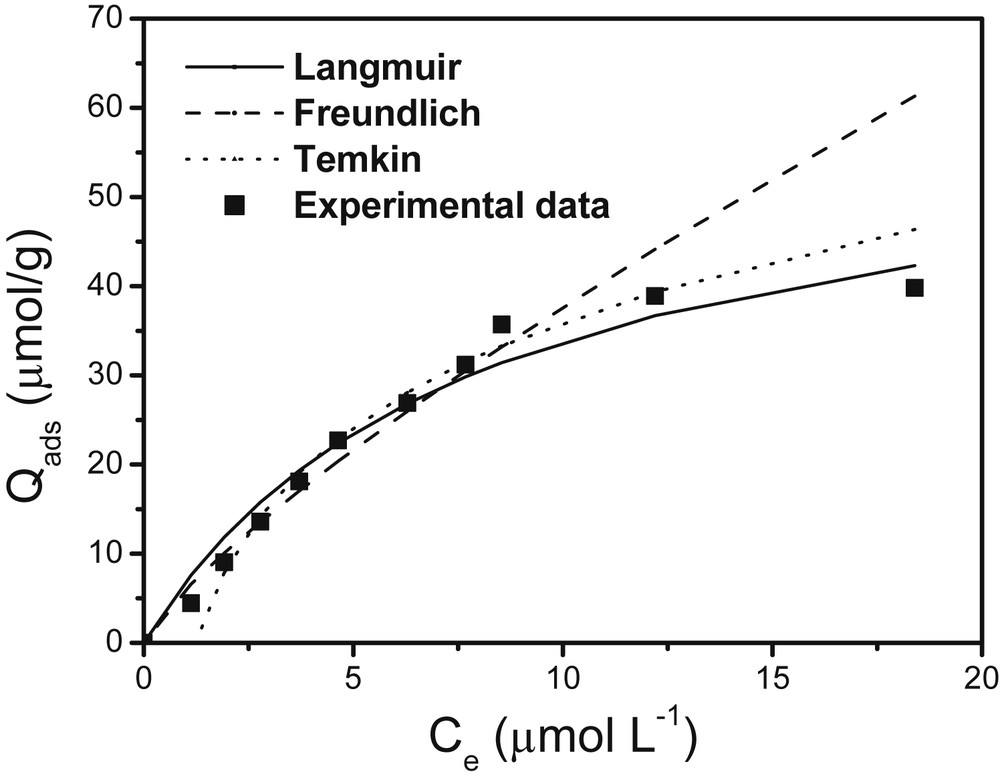
Variation of the concentration of Cu(II) ions sorbed at the equilibrium in solution on MSHNO3. Simulation models of Langmuir, Freundlich and Temkin. Experimental conditions: m = 20 mg; granulometry: 0–200 μm; contact time: 20 min; shaking speed: 150 rpm; V = 10 mL.
Langmuir, Freundlich and Temkin constants for the adsorption of Cu(II) on NSHNO3 and MSHNO3. Initial concentration.
Biosorbents | Isothermes types | Constants | |
NSHNO3 | Langmuir | Qm (μmol/g) | 60.386 ± 0.006 |
KL (L/μmol) | 1.088 ± 0.004 | ||
R2 | 0.994 ± 0.009 | ||
Freundlich | 1/n | 0.56 ± 0.02 | |
KF (μmol1−n.Ln. g−1) | 18.67 ± 0.02 | ||
R2 | 0.68 ± 0.03 | ||
Temkin | ΔQ (kJ/μmol) | 8.04 ± 0.02 | |
KT (L/μmol) | 1.47 ± 0.03 | ||
R2 | 0.88 ± 0.04 | ||
MSHNO3 | Langmuir | Qm (μmol/g) | 44.90 ± 0.02 |
KL (L/μmol) | 0.13 ± 0.02 | ||
R2 | 0.94 ± 0.03 | ||
Freundlich | 1/n | 0.8 ± 0.05 | |
KF (μmol1−n.Ln. g−1) | 5.97 ± 0.09 | ||
R2 | 0.97 ± 0.09 | ||
Temkin | ΔQ (kJ/μmol) | 8.78 ± 0.08 | |
KT (L/μmol) | 0.83 ± 0.02 | ||
R2 | 0.99 ± 0.02 |
A comparison of investigated models reveals that the Langmuir model presents a better correlation coefficient (R2 = 0.994) for the NSHNO3. This implies that the NSHNO3 sorption sites display an equivalent energy and are uniformly distributed so that cupric ion fixation proceeds by the monolayer type [16,28].
The maximum sorption capacities determined by the Langmuir model were 60.386 μmol/g for NSHNO3 and 44.90 μmol/g for MSHNO3 biosorbents. Although this model is rather common for biosorption of metal ions, it is difficult to compare the qm values of various sorbents because the used experimental conditions have to be identical, what is not the case herein. Differences of metal uptake are due to the properties of each sorbent material such as the intrinsic structure, bearded functional groups and surface area. For comparison, copper(II) biosorption capacities obtained at NSHNO3 and MSHNO3 are higher than those of some other sorbent materials reported in the literature (Table 2).
Comparison of maximum capacities of some adsorbent materials for Cu(II) ion sorption, from the literature.
Adsorbent material | Qm (mg/g) | References |
Cellulose | 7.06 | [29] |
Peanut hull carbon | 65.57 | [30] |
Dried activated sludge | 62.50 | [31] |
Olive leaves powder | 71.43 | [32] |
Phanerochaete chrysosporium | 248.15 | [33] |
Pinussylvestris | 28.83 | [16] |
Globosum leaves powder | 12.45 | [11] |
Newspaper scraps | 286.83 | This work |
Maize spatha | 176.96 | This work |
In the case of MSHNO3, the Freundlich model seems to be more suitable (R2 = 0.970) than the Langmuir model (R2 = 0.941). Moreover, the sorption sites are heterogeneous and fixing energies are different [34,35]. The constant value 1/n, determined by the Freundlich model (0.8 for MSHNO3 and 0.56 for NSHNO3) between 0 and 1 is an indication of the affinity of the biosorbents for Cu(II) ions. These different values determined from this model show that the MSHNO3 material has a better affinity with Cu(II) ions, compared to NSHNO3. This phenomenon could be explained by the fact that MSHNO3 adsorption sites are more active than those of NSHNO3. This good ability of the pollutant to bind to the active sites present on the surface of MSHNO3 is confirmed with regard to the adsorption equilibrium that was attained more quickly with MSHNO3 (20 min) compared to NSHNO3 (40 min).
Temkin's model was used to determine the different heats of adsorption involved during various adsorbate–adsorbent interactions. There is a large variation of heat with the MSHNO3 (8.779 kJ/mol) compared to NSHNO3 (8.044 kJ/mol). This may reflect the fact that interactions between Cu(II) ions and MSHNO3 are more energetic than interactions between Cu(II) ions and NSHNO3 [14].
3.8 Sorption kinetics
Contact time is a fundamental parameter in all transfer phenomena of biosorption. Experiments were carried out to determine the time of equilibrium for biosorption. Both materials showed a fast rate of sorption during the first 10 min of the metal-biosorbent contact. This observation agrees with the previous other work on sorption of copper on biosorbents [13,16,36]. The equilibrium occurred within 20 min for MS and 40 min for NS. After this equilibrium period, the amount of adsorbed metal ions did not significantly change with time. The sorption kinetics was examined for better understanding of the dynamics of biosorption of Cu2+ on the biosorbents. With the number of the kinetic models which appear in the literature, the pseudo-second order and the intra-particle diffusion were applied to the experimental data obtained (Fig. 9). The modeling of the experimental kinetic data allows a description of the mechanisms of fixation, in particular by the calculation of the rate of sorption, the coefficients of mass transfer and intra-particle diffusion [37,38]. The linear and nonlinear formulae of the kinetic model of the pseudo-second order are given respectively by the following equations:
(12) |
(13) |
(14) |
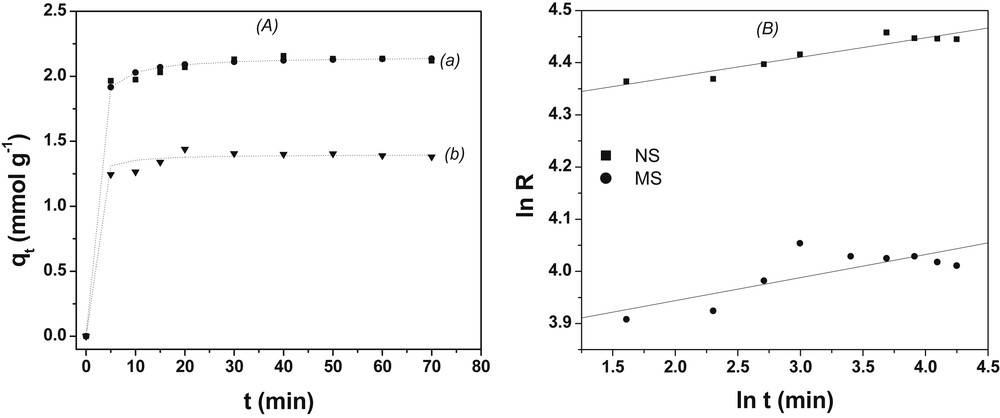
Effect of contact time on Cu(II) biosorption. (A) Pseudo second order (a) NSHNO3 and (b) MSHNO3 and (B) intra-particle diffusion kinetic models. Experimental conditions: m = 20 mg; granulometry: 0–200 μm; tMS = 20 min; tNS = 40 min vMS = 150 rpm; vNS = 100 rpm; V = 10 mL.
The intra-particle diffusion model evaluates the contribution at the diffusion of copper within biosorbents in the whole biosorption process. The model is defined by the following relationship:
(15) |
Pseudo-second order kinetic and intra-particle diffusion parameters for biosorption of copper on NS and MS.
Biosorbents | Kinetic model | Constants | |
NSHNO3 | Pseudo-second order | K2 | 0.75 ± 0.09 |
qe | 2.155 ± 0.002 | ||
R2 | 0.99 ± 0.02 | ||
t1/2 | 0.620 ± 0.001 | ||
Intraparticle diffusion | Kid | 73.55 ± 0.02 | |
α | −0.037 ± 0.005 | ||
R2 | 0.874 ± 0.001 | ||
MSHNO3 | Pseudo-second order | K2 | 2.1 ± 0.3 |
qe | 1.400 ± 0.007 | ||
R2 | 0.99 ± 0.02 | ||
t1/2 | 0.337 ± 0.002 | ||
Intraparticle diffusion | Kid | 47.229 ± 0.044 | |
α | −0.05 ± 0.02 | ||
R2 | 0.612 ± 0.001 |
The high correlation coefficient values show that the sorption data match pseudo-second order kinetics. This is generally the case for the biosorption of heavy metals or the organic compounds [17,26,32]. This is also confirmed by the values of the sorption capacity evaluated from the pseudo-second order (2.155 ± 0.002) mmol/g NSHNO3 and (1.400 ± 0.007) mmol/g MSHNO3) that are close to the experimental average values (2.157 mmol per g NSHNO3 and 1.44 mmol per g MSHNO3) obtained during the variation of contact time. This implies that the limiting step of the copper biosorption on the biosorbents is governed by chemisorption [39,40]. The reaction half time confirms that the biosorption process is very fast in the first moments on MS (0.337 min) compared with those of the NS (0.620 min).This observation shows that the sorption sites of MS although less significant seem to be more available for the uptake of cupric ions.
The intra-particle diffusion model enabled us to study the diffusion of copper within the biosorbents throughout the process. The diffusion constants show that the diffusion is very fast for NSHNO3 (Kid = 73.553 mmol/g.min1/2) compared to MS (Kid = 47.229 mmol/g.min1/2).
3.9 Desorption of copper (II) ions adsorbed
The performance or the effectiveness of a biosorbent can also be determined by its possibility to be reused. The desorption results are given in Fig. 10 where the variation of desorption percentage is plotted against concentration of the desorption solution.
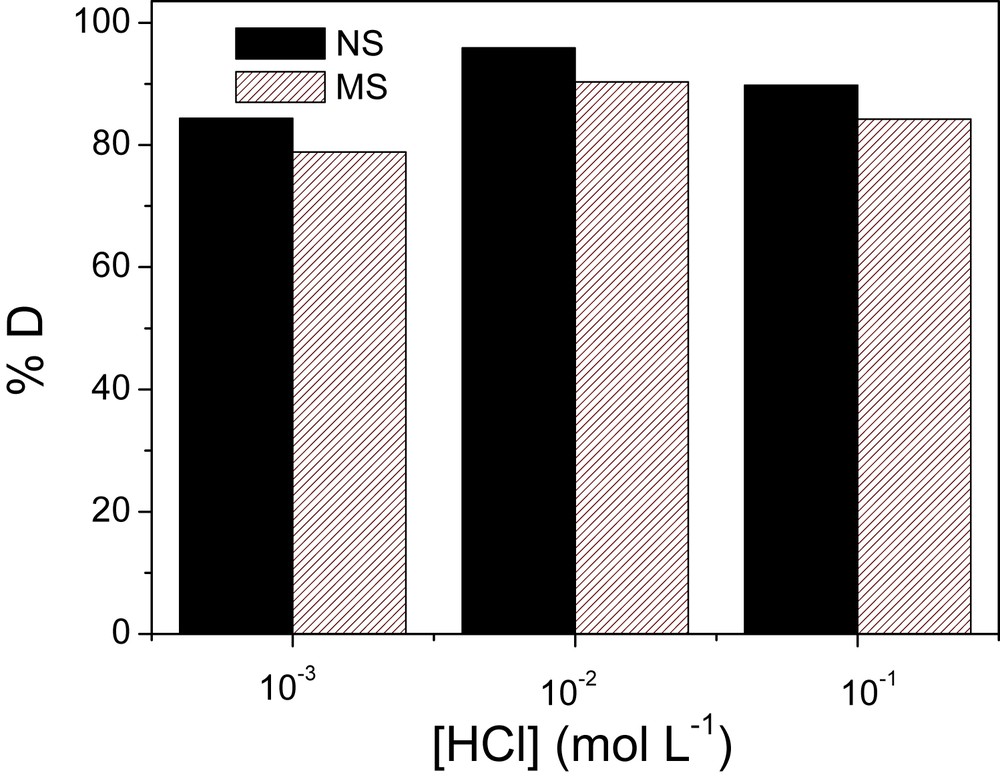
Effect of the desorption solution HCl (0.001, 0.01 and 0.1 M) on the recovery of sorbed Cu(II) ions on NSHNO3 and MSHNO3. Experimental conditions: m = 20 mg (containing copper), Contact time: 60 min, shaking speed: 200 rpm, V = 10 mL of HCl.
The desorption percentage obtained for the various concentrations of HCl (0.001–0.1 M) lies between 84.38 and 95.88% for NS and 78.84 and 90.34% for the MSHNO3. Therefore, the NSHNO3 have a capacity more significant to release the copper ions compared to the MSHNO3. The undesorbed fraction of copper may be bound to the NS and MS through a different mechanism other than physical sorption. The desorption percentage of NSHNO3 and MSHNO3 is 84.38 and 78.84%, when the concentration of HCl is 0.001 M and 95.88 and 90.34% when the concentration of HCl is 0.01 M, respectively. Then, it is clear that the desorption results from cation exchange between the protons in solution and the sorbed cupric ions. The action of the protons only starts effectively from a certain concentration of HCl. The desorption process even slows down for higher concentrations of HCl (0.1 M), in the order of 89.78%–84.24% for the NS and MS, respectively. This can be explained by the fact that for such concentrations, the hydrochloric acid could modify the structure of the materials, increasing the affinity between the cupric ions and the biosorbent [14,22,37].
4 Conclusion
The ability of two natural biosorbents (newspaper scraps (NS) and maize spatha (MS)) for the removal of copper(II) ions from aqueous solution was evaluated. An optimization of the parameters affecting the rate of removal and the mechanisms that take place during the biosorption of copper ions were investigated. It was noticed that to achieve sorption capacities, optimal conditions should be as follows: Cu(II) ion concentration: 5 × 10−3 M, contact time: 40 min for NSHNO3 and 20 min for MSHNO3, pH 5, shaking speed: 100 rpm for NSHNO3 and 150 rpm for MSHNO3, and biosorbent dosage: 2 g/L. The sorption isotherms showed that the distribution of the sorption sites on the surface of NSHNO3 is homogeneous and heterogeneous for MSHNO3. The sorption kinetics of both materials is best described by the pseudo-second order model. The interfering ions in solution decreased significantly the sorption capacity of the cupric ions. Furthermore, the stripping medium (0.01 M HCl) used allows to consider the reuse of the investigated biosorbents (a desorption rate of 95.8% for NSHNO3 and 90.34% for MSHNO3). The promising results obtained throughout this work showed that both materials are important sorbents useful for the treatment of water polluted by Cu(II) ions.
Acknowledgements
The authors acknowledge the support of the Academy of Science for the Developing World (Grant No. 07-052-LDC/CHE/AF/AC).