1 Introduction
The field of bone tissue engineering (BTE) [1,2] was initiated three decades ago to induce new functional bone regeneration [3]. Engineered bone grafts have been shown to have capacity for osteogenesis, osteoconduction, osteoinduction, and osteointegration [4]. In this context, a wide range of materials have been developed and identified as biomaterials for BTE. These materials can be classified into three categories: natural [5] or synthetic polymers [6], ceramics such as calcium phosphates and bioactive glasses or glass ceramics, and composites [7]. Despite the huge research efforts worldwide in this field, it is difficult to obtain a biomaterial able to satisfy most of the requirements need for effective bone regeneration. A better choice for BTE is to use a composite system [8,9], which confers not only mechanical properties but also osteoconduction and osteointegration.
Given its chemical similarity to the main structural proteins found in the extracellular matrix of several hard tissues, type I collagen has been tested in bone regenerative studies because of its ability to replicate the three-dimensional bone structure [10]. It facilitates a natural biological potential by creating a favorable environment for cellular adhesion, cellular migration, and cell growth [11]. Chemical cross-linking of collagen can modify its mechanical and physical properties, and also composite system of collagen and ceramic improves its mechanical properties and enhances bone conductivity [12,13].
On the other hand, hydroxyapatite (HA, Ca10(PO4)6(OH)2), one of few materials classified as bioactive, osteoconductive, and biocompatible with hard tissues, is widely used in dental implants. Different chemical procedures for HA synthesis use precursors such as CaCO3 [14], Ca(NO3)2, or Ca(OH)2 as the source of calcium ions and (NH4)2HPO4, H3PO4, or Na2HPO4 as the source of phosphate ions. However, commercially available HA is relatively expensive because of the use of high purity reagents. The HA derived from natural materials provided by marine organisms such as corals [15], seashells [16], eggshells [17], snails [18] has an important advantage like better tissue response owing to its chemical and structural similarity with the inorganic constituents of biological hard tissues and strong bonds with the hard tissues.
It is known that a part of marine species, like corals, is limited and protected, whereas, on the contrary, there are a variety of available and abundant materials that have not been used for different applications till now. The worldwide availability, the low production cost of Rapana thomasiana (RT) shell [19], its biological-natural origin, and mechanical properties similar to human bone are important characteristics that classify this shell as an ideal candidate for preparing calcium phosphate materials used in biomedicine. RT, a predatory gastropod considered an environmental threat [20] contains 95–99% by weight of CaCO3, which allows to be used in various applications. Several studies have been previously reported for synthesis of collagen/HA composites for BTE.
The present article reports a newly developed method for obtaining a highly porous collagen/HA composite for bone tissue regeneration. This new biomaterial produced in the form of sponge is obtained from collagen gel (CG) combined with CaCO3 originated from recycled RT seashells and Na2HPO4·2H2O. In this case, the collagen acts as a matrix for diffusion of calcium and phosphate ions to perform in situ HA synthesis. To confirm the conversion of the recycled seashell of RT into HA, a comparative CG/commercial HA sponge was prepared. The differences between biomaterial characteristics and their potential application have been examined by spectroscopic study and surface morphology investigations. Also, in vitro studies have been performed with MG63 human osteoblasts to establish the cell behavior in terms of cell survival and proliferation status.
2 Experimental part
2.1 Materials
Stranded individuals of RT were collected from the beach of Cape Midia (N44.34507°, E28.69201°), Black Sea, Romania. Type I CG of bovine origin with an initial concentration of 2.11% and approximate pH of 2.3 was provided by Collagen Department of National Research & Development Institute for Textiles and Leather, Bucharest, Romania. Glutaraldehyde (GA; Merck, Germany) as a cross-linking agent and commercial Fluka HA were used. Na2HPO4·2H2O (Sigma-Aldrich Co., Germany) as a source of phosphate ions and commercial Fluka HA (Sigma-Aldrich Co., Germany) were used. Analytical grade NaOH 1 M was used for the pH adjustment.
2.2 RT shell preparation
The RT shell was first washed in distilled water and dried in oven at a temperature of 105 °C. The fragments obtained were milled using a pestle and a mortar followed by ball milling with zirconium oxide balls (Retsch GmbH, Haan, Germany) in a horizontal ball mill (9VS; Pascall Engineering Co. Ltd, Suffolk, UK) for 6 h. The shell powder (CaCO3) was left for 3 h in acetone solution to remove the organic matter and afterward rinsed with distilled water, oven dried at 105 °C for 1 h, and sieved in the Analysette 3-pro shaker (Fritsch GmbH, Germany) to segregate the particle to specific sizes less than 45 μm.
2.3 Preparation of marine organism and natural polymer based biomaterials
The RT powder (< 45 μm) was added to the CG and mixed under stirring for 5 h at 25 °C, with a required volume of an aqueous solution of Na2HPO4·2H2O to set the stoichiometric molar ratio of Ca/P equal to 1.667 appropriate for HA formation. The main conversion is running according to the following reaction:
The increase in temperature and the low pH of the CG promoted the binding between collagen fibers and HA, which was obtained in situ through the precipitation of CaCO3 microparticles in Na2HPO4·2H2O solution. One percent of a GA solution was added to facilitate cross-linking and was mixed for another 60 min at 25 °C. The obtained homogenous mixture with an adjusted pH between 7.4 and 7.6 was transferred to a well culture plate and prefrozen at −15 °C for 24 h and freeze-dried at 0.12 mbar starting from −40 to +30 °C during 40 h to obtain the porous HA/composite biomaterial. The resultant sponge (sample A) had a form of a disc with a diameter of 15 mm and thickness of 10 mm (Fig. 1).
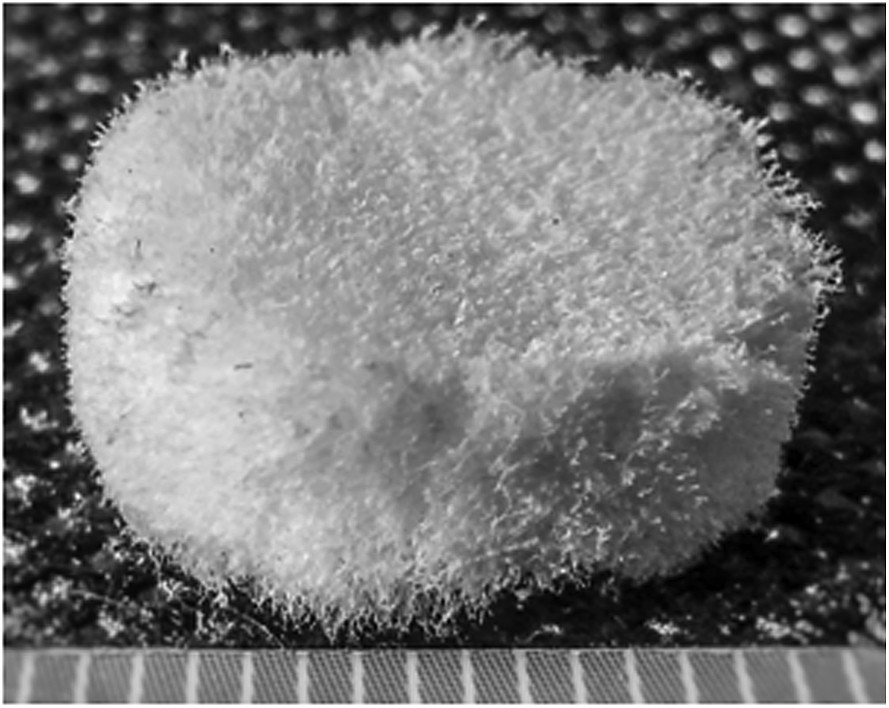
Macroscopic profile of composite biomaterial A – CG/RT and Na2HPO4·2H2O.
A similar type of sponge (sample B) based on commercial synthetic HA and CG was prepared by using the same procedure. For reference, a sponge based on CG without any ceramic material was prepared (sample C).
The gravimetric ratio between dry substance of CG and HA, or between CG and RT with Na2HPO4·2H2O was 1:5, and 0.5% between GA and CG with HA, or between GA and CG with RT and Na2HPO4·2H2O.
2.4 Characterization techniques
Both raw materials and newly prepared biomaterials were characterized for their composition and morphology. Fourier transform infrared (FT-IR) spectroscopy was performed using a Perkin Elmer Spectrum 100 FT-IR spectrophotometer in the attenuated total reflection mode. The spectra were collected in the range 4000–600 cm−1 at a resolution of 4 cm−1, and 16 repeated scans were averaged for each spectrum. Surface morphology of the new sponges was examined by scanning electron microscope (SEM) analysis. To increase the conduction electron beam, the samples were coated with Ag before examining with a Hitachi S2600N SEM.
2.5 Cell culture
The biological behavior of the cells in contact with the composite scaffolds CG/HA was tested in terms of cytotoxicity/viability and proliferation using a human osteoblast-like cell line, MG63, derived from human osteosarcoma. Osteoblasts were cultured in Dulbecco's Modified Eagle Medium supplemented with 10% fetal bovine serum and 1% penicillin/streptomycin and maintained in standard conditions at 37 °C in a humidified 5% CO2 atmosphere. Cells were detached by treatment with trypsin–EDTA (0.25% and 0.53 mM, respectively) and seeded, at an initial density of 5 × 104 cells/cm2, on the top of disk-shaped composite scaffolds placed into 24-well plates. The resulted cell-scaffold bioconstructs were maintained in standard culture conditions for 2, 4 and 6 days. The medium was exchanged every second day and all experiments have been performed in triplicate.
2.6 Lactate dehydrogenase cytotoxicity assay
Cytotoxic potential of the composite scaffolds was evaluated by the quantification of lactate dehydrogenase (LDH) released in the culture medium by osteoblasts grown on the scaffolds. LDH assay was performed after 2, 4, and 6 days of culture by using “LDH-based In Vitro Toxicology Assay Kit” (Sigma-Aldrich Co.) according to the manufacturer's protocol. Briefly, the media were harvested and mixed with the solutions provided in the kit and after the incubation time, LDH activity was determined by measuring the optical density of the resulting solution at 490 nm using an Appliskan Thermo Scientific reader.
2.7 MTT viability and proliferation assay
The viability and proliferation of MG 63 osteoblasts cultured onto the composite scaffolds was quantitatively determined at 2, 4, and 6 days postseeding using 1 mg/mL of 3-(4,5-dimethylthiazol-2-yl)-2,5-diphenyltetrazolium bromide (MTT, Sigma-Aldrich Co., M 2185), as previously reported [21]. The amount of formazan produced by metabolically active viable cells was recorded at 550 nm using a microplate reader (ThermoScientific Appliskan).
2.8 Statistical analysis
Triplicate specimens were used for LDH and MTT assays to ensure the reproducibility of the results, and statistical analysis was performed with GraphPad Prism software using one-way analysis of variance with Bonferroni's multiple comparison tests. The results are presented as the means ± SD (standard deviation). The P values < 0.05 were considered to be statistically significant.
3 Results and discussion
3.1 Infrared spectroscopic study
Infrared spectroscopy is an efficient tool for the investigation of chemical composition of different components and interactions among the components. To understand the interactions between collagen and ceramic material, the FT-IR study was started by recording the FT-IR spectrum of pure CG in the form of sponge (sample C) (Fig. 2). The spectrum of CG shows typical amide bands as following: the band at 1633 cm−1 assigned to amide I, specific for CO stretch in collagen; the bands at 1548 and 1237 cm−1 assigned to amide II and amide III, respectively, corresponding to in-plane deformation of NH and to the stretching vibration of CN, respectively. Other typical bands, such as stretching vibration of NH at 3296 cm−1 for the amide A and the stretching vibration of CH at 3075 cm−1 for the amide B, are evident in the spectrum [22,23].
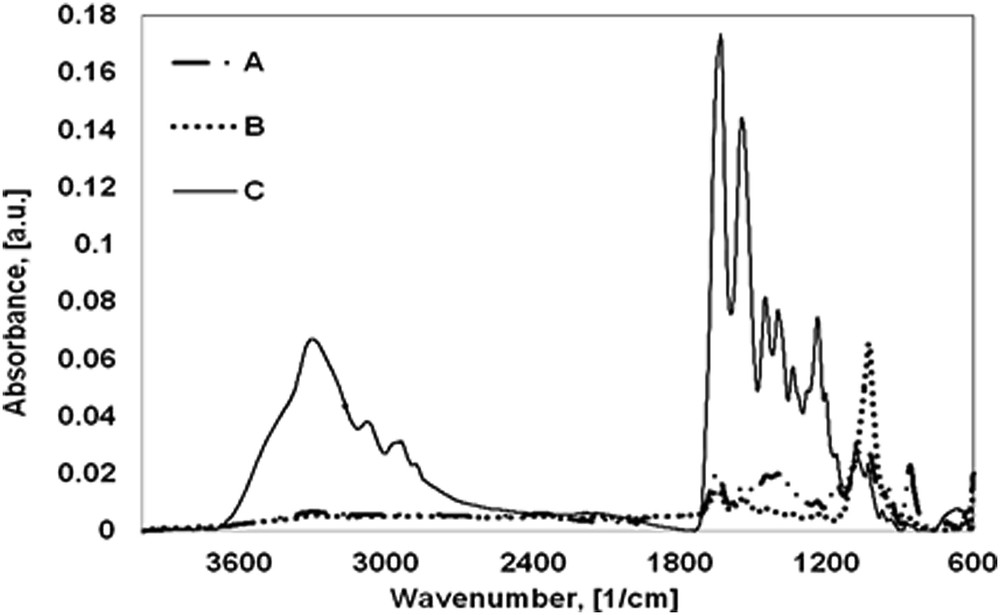
FT-IR spectra of new composite biomaterials: A – CG/RT and Na2HPO4·2H2O; B – CG/commercial HA; and C – CG.
The spectrum of RT shell exhibited the bands at 1463, 861, and 711 cm−1 characteristic for υ3, υ2, and υ1 vibrations of the carbonate anion of CaCO3 as calcite [24]. Its fingerprint is represented by the most intensive band at υ3 because it is a double degenerated vibration. The organic constituents from shell are below the detection limit of IR spectra. Similarly, the FT-IR spectrum of pure commercial HA exhibits typical peaks of phosphate and hydroxyl anions. The small absorption band is present at around 3566 cm−1 because of the stretching mode of hydroxyl group. Two bands at 1026 and 962 cm−1 associated with the asymmetric and symmetric stretching of the PO bond form , respectively, and a band at 633 cm−1 because of the bending mode of PO in is present.
The composition of the marine organism and natural polymer based biomaterials becomes very clear when its FT-IR spectrum (Fig. 2A) is compared with those of its components.
FT-IR spectra of samples A and B show typical bands for CG such as amide I, II, and III bands at around 1650, 1540, and 1233 cm−1. The shifting of the main amide peak of collagen (1633 cm−1) from sample C to a higher wavenumber for the sample A (1652 cm−1) and sample B (1648 cm−1) is probably because of the Ca2+ from HA and CO from collagen interaction. In addition to the important peaks of CG, the spectra of new biomaterials exhibited characteristic bands from HA. Most of the absorptions from phosphate vibrations are observed both in the spectra of samples A and B and of raw commercial HA, which confirms the formation of HA in sample A based on CG and RT shell. The absorption peaks at 1029 and 949 cm−1 in sample A and at 1029 and 962 cm−1 in sample B correspond to the asymmetric and symmetric stretching of phosphate, respectively. Also, the band at around 630 cm−1 because of the bending mode of PO in is present in both new biomaterials.
3.2 Surface morphology
SEM images shown in Fig. 3 illustrate the morphology of the biomaterials processed as sponges for bone grafting. At 100× magnification (Fig. 3 upper row) the morphology of all three samples showed a relatively dense fibrous structure because of the collagen matrix. For a better illustration of this aspect, separate SEM images were taken for the sponge based on CG (sample C).
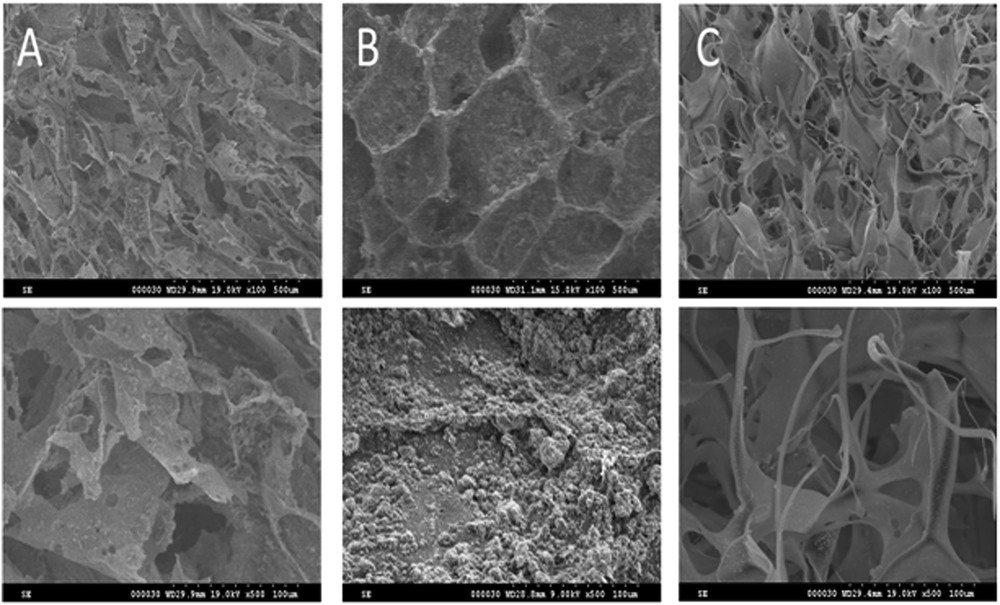
SEM images of composite biomaterials: A – CG/RT and Na2HPO4·2H2O; B – CG/commercial HA; and C – CG.
Obvious differences among samples can be noticed at 500× magnification (Fig. 3 lower row). Thus, in the microscopy images of sample A (CG/RT and Na2HPO4·2H2O), the collagen fibers appeared to be intercalated with HA derived from RT shell, whereas in the case of sample B (CG/commercial HA), the sponge appears to be covered by a HA layer with a uniform distribution. This confirms that the new method of in situ HA synthesis starting from CaCO3 originated from recycled RT seashells and Na2HPO4·2H2O into a collagen matrix represents a viable way of obtaining high quality bone substitute material.
The SEM images display an open pore structure with a pore size varying from 50 to 350 μm and pore interconnectivity. These properties are appropriate for good cell migration, growth, and accommodation during tissue regeneration process [1,25].
3.3 Evaluation of composite scaffolds' cytotoxicity
LDH is an oxidoreductase enzyme that catalyzes the interconversion of pyruvate and lactate. LDH activity is normally present in all of the body's cells being invariably found in the cytoplasm. In response to cell damage, LDH is released from the cells in the culture medium [26].
Thus, in our study, we first investigated the LDH release in the culture medium at 2, 4, and 6 days postseeding to establish if the analyzed scaffolds influenced the cellular survival. This quantitative method gave us information about the cytotoxicity of the analyzed scaffolds as it represents an indicator of cell membrane integrity. As shown in Fig. 4, the MG 63 osteoblasts seeded onto all analyzed scaffolds displayed low LDH release in culture medium without significant differences at 2 days of culture. Higher values for LDH levels were remarked for CG/RT and Na2HPO4·2H2O (P < 0.01) composite scaffolds comparatively with CG/commercial synthetic HA and CG after 4 days of culture. The significantly higher level of LDH was also observed at 6 days of culture for CG/RT and Na2HPO4·2H2O (P < 0.001) and CG/commercial synthetic HA (P < 0.01) comparatively with CG scaffold. However, this increase in LDH levels can be explained by the possible cell death that could appear as the cell confluence proceeds on composite scaffold surfaces.
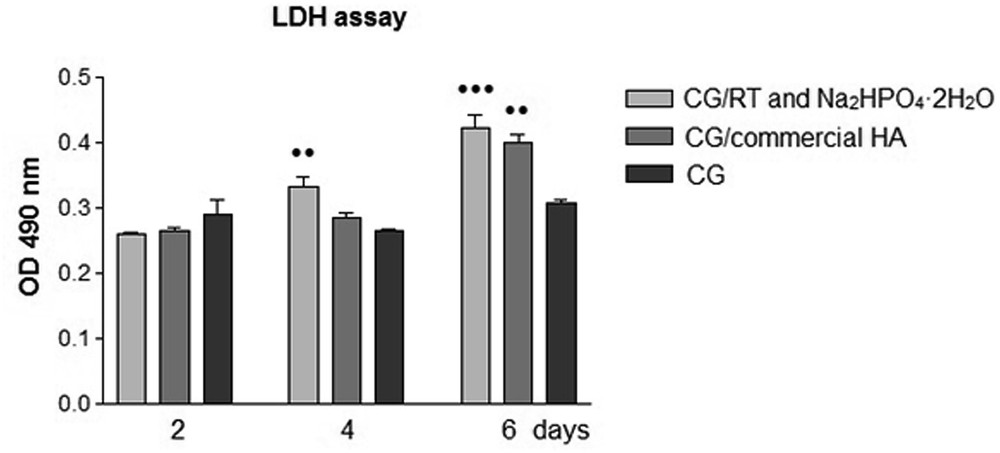
Comparative evaluation of LDH activity released into the media by MG 63 cells grown onto the composite scaffolds and CG at 2, 4, and 6 days postseeding. Results are presented as the means ± SD (n = 3), [•• P < 0.01 (CG/RT and Na2HPO4·2H2O vs CG/commercial HA and CG at 4 days); •• P < 0.01 (CG/commercial HA and CG at 6 days); and ••• P < 0.001 (CG/RT and Na2HPO4·2H2O vs CG at 6 days)]. OD, optical density.
3.4 Viability and proliferation status of MG63 osteoblasts on the developed scaffolds
Another important objective of biological studies was to investigate the MG 63 viability and proliferation rate onto analyzed composite scaffolds using an MTT reduction assay, which is very important because it provides information about the cell growth and the metabolic activity of the cells. It might be noticed (Fig. 5) that the levels of MTT conversion increase in time, showing an increasing number of viable cells as a result of the active cell proliferation for more than the 6-day incubation period.
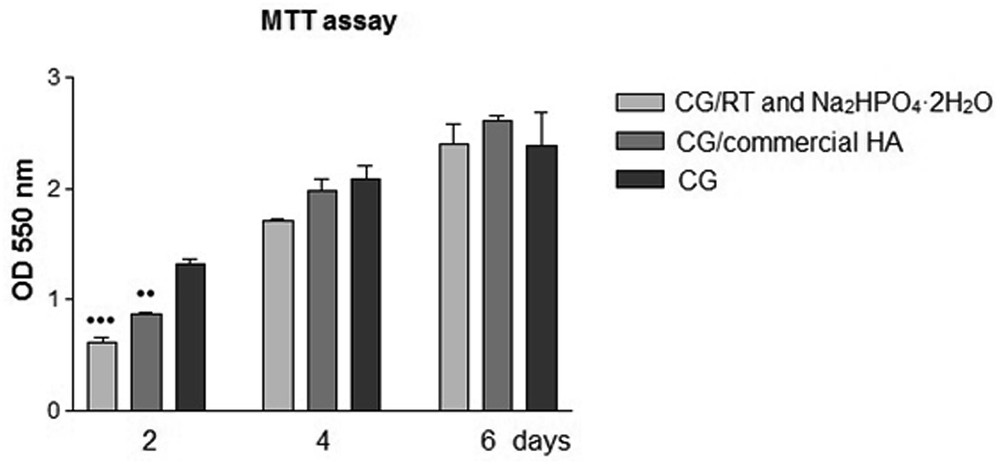
Viability and proliferation rate of MG63 osteoblast cultured onto composite scaffolds and CG for 2, 4, and 6 days was determined by MTT assays. Data analysis was based on the mean ± SD (n = 3). [•• P < 0.01 (CG/commercial HA and CG at 2 days) and ••• P < 0.001 (CG/RT and Na2HPO4·2H2O vs CG at 2 days)]. OD, optical density.
At 2 days postseeding, statistically significant differences in cell viability and proliferation rate were observed between CG/RT and Na2HPO4·2H2O scaffolds versus CG (P < 0.001) and CG/commercial HA scaffolds versus CG (P < 0.001). This cell behavior could be explained by a short period of time that the cells need to accommodate to the new environment provided by the composite scaffolds based on collagen and HA. Thus, at 4 and 6 days postseeding, no statistically significant differences in cell viability and proliferation were observed between samples.
4 Conclusion
The present article reports the elaboration of a new method for the obtaining biomaterials based on natural polymer and marine organism, in particular RT shells, for BTE. The conversion of CaCO3 from recycled RT seashells into HA was confirmed by FT-IR spectra and by SEM images of the obtained biomaterials. This new method of in situ conversion of CaCO3 microparticles into HA exhibits good binding qualities between collagen and HA, thus offering a stable matrix structure. The success of using these new biomaterials based on collagen and ceramic material as sponges for bone tissue regeneration is related to the fact that this composite is biocompatible, forming a favorable matrix for cells growth and proliferation because of the advantage of collagen osteoinduction and bioactivity and osteoconduction of HA. The biological experimental data obtained with MG63 osteoblasts seeded onto developed scaffolds suggest that the composite scaffolds support cellular survival and proliferation. These findings make the analyzed samples possible candidates for BTE.