1. Introduction
Enzymes, nature’s catalysts, have evolved to facilitate biochemical reactions with remarkable specificity. Historically, they have been harnessed in fermentation processes for food and beverage production [1, 2, 3, 4], and early industrial applications were predominantly focused on the use of naturally occurring enzymes like xylose isomerase for high-fructose corn syrup production [5], nitrile hydratase for acrylamide synthesis [6, 7], and penicillin amidase for semisynthetic penicillins such as ampicillin [8]. Their high selectivity avoided the need for complex protective group strategies, thereby simplifying processes, reducing waste, and aligning with the increasing demand for sustainable industrial practices.
Despite these examples, natural enzymes often exhibited limitations for industrial-scale application. Their narrow substrate scope and suboptimal operational stability in intensified conditions often hindered broader industrial adoption [2]. Advancements in directed evolution [9, 10] and protein engineering [11] have significantly mitigated these limitations. There are examples of evolved enzymes with enhanced solvent resistance [12], improved stability under process conditions, optimized pH and temperature ranges, and even more importantly, where their reactivity is tailored to the substrate of interest while maintaining excellent enantioselectivity [13].
In parallel to the growth of biocatalysis, flow chemistry has become increasingly important over the past few decades. Unlike traditional batch processes, flow chemistry allows for more precise control of the reaction conditions (temperature, pressure, contact time between substrate and catalyst), resulting in higher yields, safer operations, and reduced waste [14, 15, 16]. Recently, the interest in flow strategies has grown significantly in the fine chemical industry [17, 18, 19, 20, 21]. Among the multiple reactor types, packed-bed reactors (PBRs) allow the confinement of the catalyst onto a column or similar reactor type. The confinement of the catalyst ensures a clean out-stream, facilitating downstream processing and product purification. Moreover, while scale-up is possible, PBRs also allow for the scale-out strategy—the use of parallel small-scale reactors to process larger volumes in shorter times. For this, PBRs hold exciting potential in the synthesis optimization of fine chemicals, while reducing costs and performing consistently, in a more sustainable manner, making flow chemistry essential for modern, sustainable chemical production.
Looking at the advantages of both technologies, it is only logical to combine them; this is the objective of flow biocatalysis. However, even engineered enzymes have a major downside for their integration into continuous manufacturing: their reusability. Enzymes are water-soluble and act in solution, and due to their complex structure, their recycling and reuse for multiple batches is extremely difficult—if not impossible—without modification. To solve this problem, enzyme immobilization is crucial to enabling enzymes in continuous reactors (Figure 1). Immobilization is a simple yet extremely powerful technique that involves confining enzymes within or onto solid materials, creating a heterogeneous biocatalyst that is no longer in solution. Immobilized enzymes allow for their reuse, and enhance the cost-efficiency of enzymatic processes [3, 22]. Importantly, immobilized enzymes often exhibit enhanced stability due to the reduced flexibility upon immobilization and can retain activity over extended periods of time, even under conditions that normally would inactivate enzymes in solution [3, 23]. Immobilized enzymes have been used in the pharmaceutical, fine chemical, and food industries, and yet only a few examples of their use in PBRs are available.
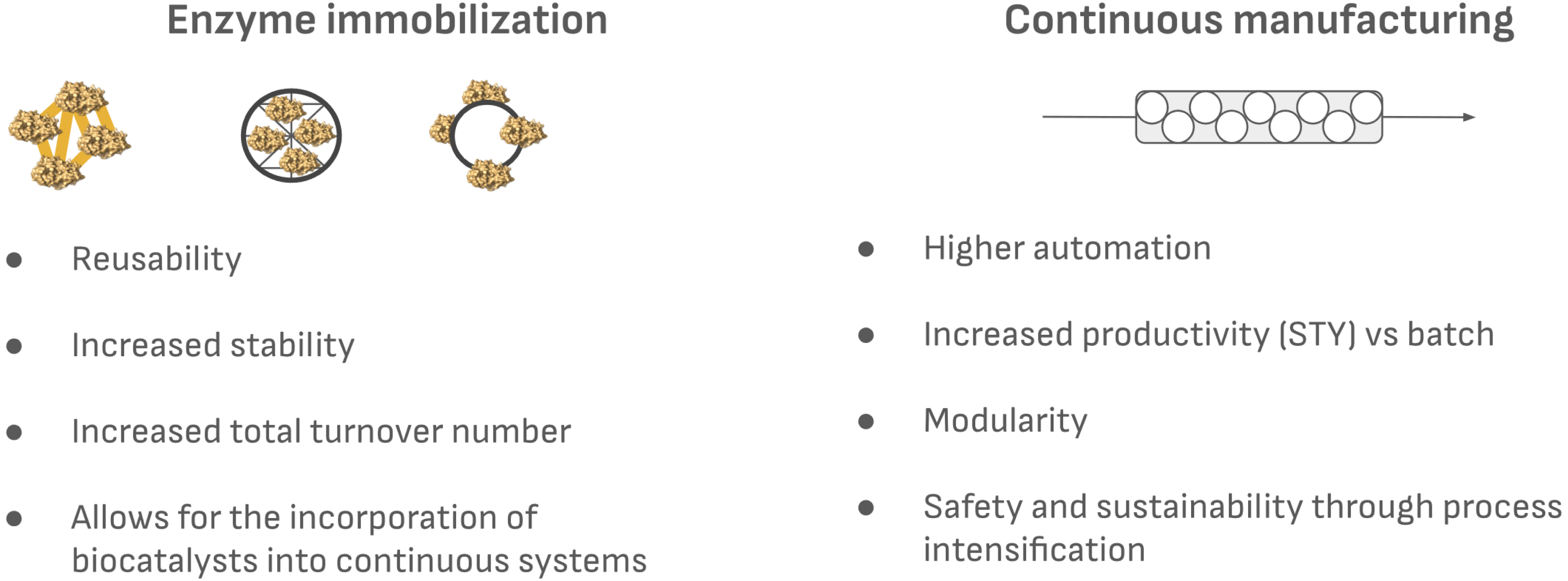
Main advantages of enzyme immobilization and continuous manufacturing.
Several reviews have addressed the evolution of flow biocatalysis during the past 15 years [24, 25, 26, 27]. In this concise review, we have focused on the applications of distinct enzyme immobilization techniques in the form of PBRs. We discuss the different immobilization methods and highlight their applications in industrially relevant transformation in continuous flow, discussing their advantages and the challenges encountered. By understanding these developments, we aim to highlight the potential for future innovations that can further integrate enzymatic processes into continuous manufacturing for industrial chemistry.
2. Immobilization techniques and their application in continuous-flow setups
Since the discovery of enzyme immobilization by Nelson and Griffith [28], many different approaches have been developed for the immobilization of enzymes. Finding a universal method has been proven challenging due to the complex nature of the enzymes and the multiple factors that play a role in the final activity and stability of the final biocatalyst. Thus, in most cases, the immobilization of choice is tailored to a specific enzyme for a desired application.
Immobilization methods are typically classified into reversible techniques (such as adsorption, encapsulation, and reversible covalent binding) and irreversible techniques (including cross-linking and irreversible covalent binding). However, for the purpose of this short overview, we classify immobilization methods based on the use (or not) of a solid support to immobilize the enzyme: carrier-based and carrier-free methods (Figure 2).
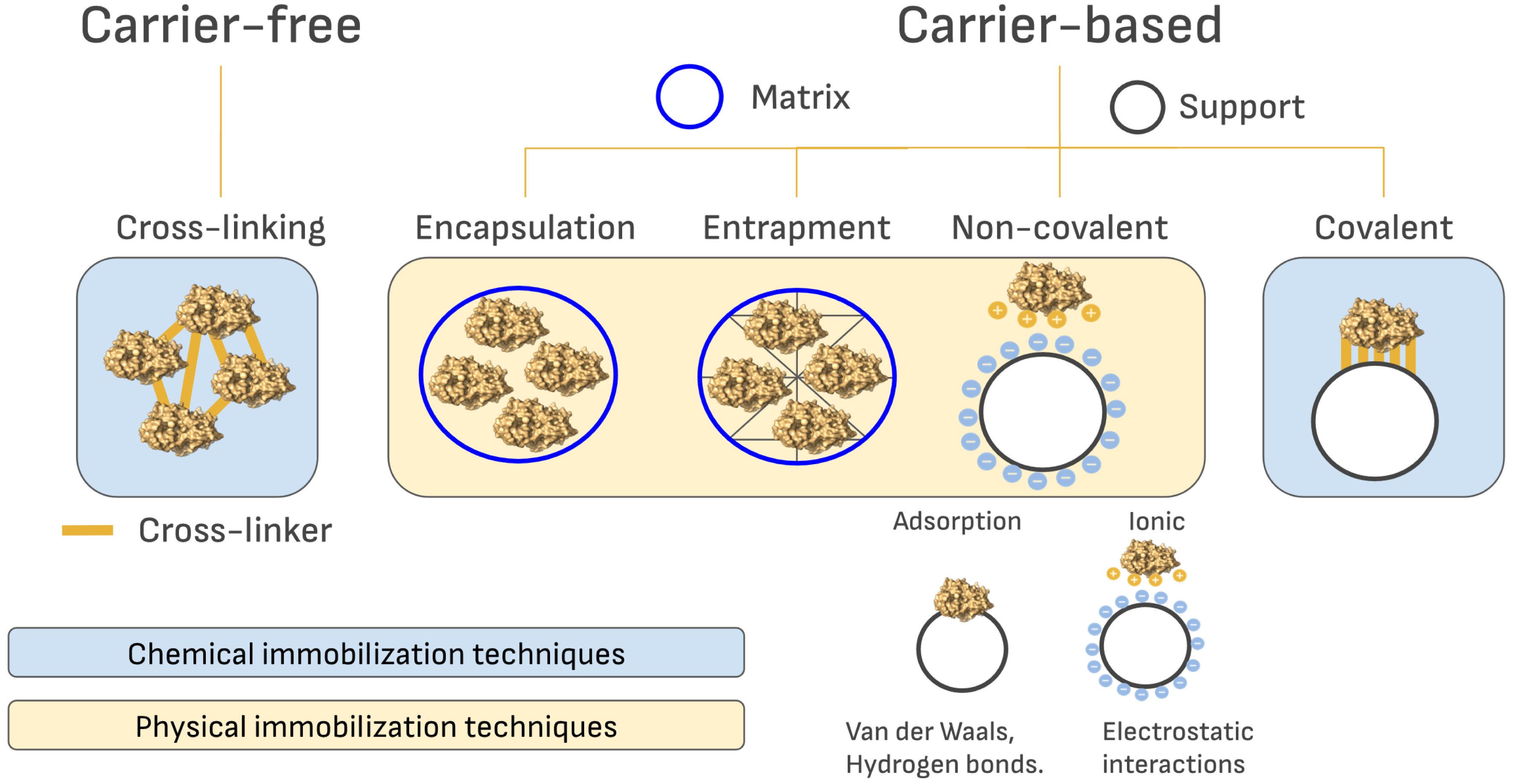
Schematic representation of the different methods of immobilization that have been used for continuous-flow biocatalysis.
2.1. Carrier-free immobilized enzymes
Carrier-free immobilization of enzymes is carried out through their cross-linking to form insoluble supramolecular structures. Cross-linking involves the formation of covalent bonds between enzymes using bifunctional or multifunctional reagents like glutaraldehyde. These methods aim to maximize enzyme loading on the final biocatalyst by eliminating the need for carriers and maximizing the activity per unit of mass. However, they also present significant drawbacks that limit their applicability, especially, their mechanical stability and reusability [29, 30].
There are different approaches to the creation of cross-linked (CL) biocatalysts. Enzymes can be cross-linked in different forms: as soluble enzymes (CLEs), lyophilized (CLELs), or in the form of enzyme aggregates (cross-linked enzyme aggregates [CLEAs]) and enzyme crystals (CLECs) [30]. Among these options, the most common strategies are CLEAs and CLECs, but only CLECs have been successfully incorporated into PBRs without further modification [31]. The application of CLEAs in flow biocatalysis has been challenging due to two main issues: the scalability in their production and their mechanical fragility [31, 32, 33]. Nonetheless, there are some examples such as the γ-lactamase CLEAs employed in a flow microreactor built from a capillary column [34, 35].
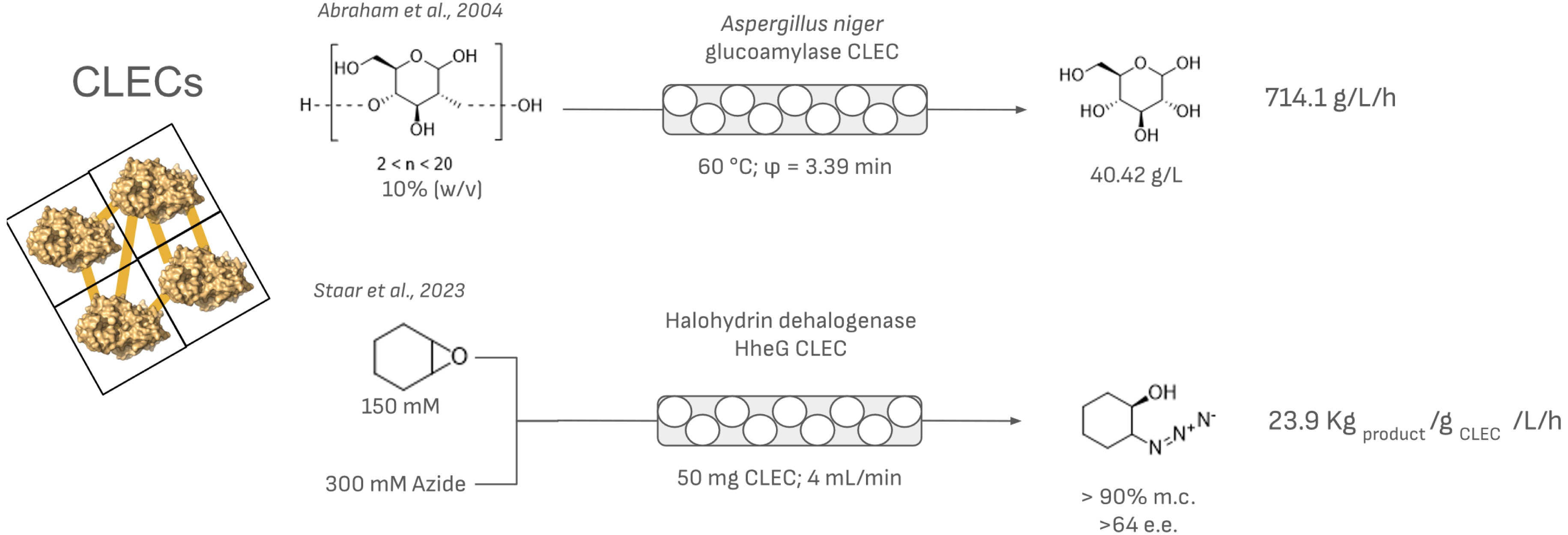
Selected examples of the use of CLECs in PBR systems.
On the other hand, CLECs show higher mechanical resistance and have been applied in different chemical reactor configurations [36, 37]. Although only a few examples are available, their higher mechanical stability has allowed their successful use in PBRs (Figure 3). For example, glucoamylase CLECs have been used for the continuous production of glucose from starch and maltodextrin in PBRs [38]. In a more recent example, the preparation of different β-substituted alcohols using halohydrin dehalogenase HheG CLECs has been successfully scaled up [32]. The production of HheG CLECs was performed using a stirred crystallization approach, enabling upscaling beyond 50 mL. The packing of these CLECs in a PBR facilitated the production of 2-azidocyclohexan-1-ol with an impressive space–time yield of 28.7 kg⋅L−1⋅day−1, demonstrating excellent operational stability and efficiency, which highlights their potential for industrial applications in continuous-flow processes.
2.2. Carrier-based methods
Carrier-based methods involve the use of a matrix or solid support that interacts with the enzymes and produces water-insoluble materials. The range of materials that have been already used is broad and expanding, including natural polymers, liposomes, petroleum-based polymers, inorganic materials, and more recently, metal-organic frameworks. The interaction of the enzyme with the carrier can be through physical confinement (entrapment and encapsulation), non-covalent interactions (adsorption, ionic interactions), or covalent bonding. Each of the strategies has its benefits and downsides. When using either entrapment or non-covalent interactions, the activity tends to be unaffected, but the reusability and stability of the resulting biocatalyst are generally low, except in specific enzyme types such as lipases, making its incorporation into continuous manufacturing processes challenging. On the other hand, with covalent methods, the reduced flexibility of the enzyme affects its activity but offers preparations with much higher stability and reusability.
2.2.1. Entrapment and encapsulation methods
Entrapment and encapsulation methods leverage a physical restriction of the enzymes or cells within a confined space or network using a carrier, in this case, a matrix. Several reviews focus on the techniques and discuss the applicability and limitations of these methods [39, 40]. In general, entrapment provides a protective environment for the enzyme, but the presence of a surrounding extra layer causes diffusional limitations. Moreover, if the pore size of the matrix is bigger than the enzyme size, potential leakage might happen during the reaction.
Probably the most common method of encapsulation of enzymes is alginate hydrogels [41, 42]. Since the 1990s, a lot of research has focused on this method for its easy, cost-efficient, and mild conditions for preparation. Carefully controlling the conditions of polymerization, precursors, and modifiers allows for tailoring material porosity, which is especially important. Nonetheless, alginate encapsulation often suffers from low recyclability, a key aspect for applying biocatalysis in PBRs. Two different approaches have been taken to solve these problems: a post-entrapment treatment of the alginate beads and the entrapment of supramolecular enzyme structures (CLEAs, CLECs, and others).
One of the simplest post-modifications to alginate beads that has shown advantages is their drying. The so-called dried alginate-entrapped enzymes have been used in continuous flow for the production of fructo-oligosaccharides starting from sucrose (Figure 4). This strategy yielded a process with excellent productivity with a cheap and cost-effective immobilization method while keeping enzyme leakage minimal by selecting the appropriate process conditions (especially the control of water content in reaction to avoid rehydration) [43]. More recently, coating strategies with various polymers have also been tested to stabilize the alginate beads further, increase their reusability, and prevent the leaching of the enzyme. In some cases, the resulting preparations have been applied in PBRs, mostly as proof-of-concept reactions to show their applicability and advantages in terms of stability and reusability [44, 45]. Although these strategies have been successful, the addition of a second layer hampers the free diffusion of the substrate and product from the bulk of the reaction to the biocatalyst. On the other hand, there are only a few examples of the use of supramolecular enzyme structures entrapped in alginate in PBRs [46, 47].
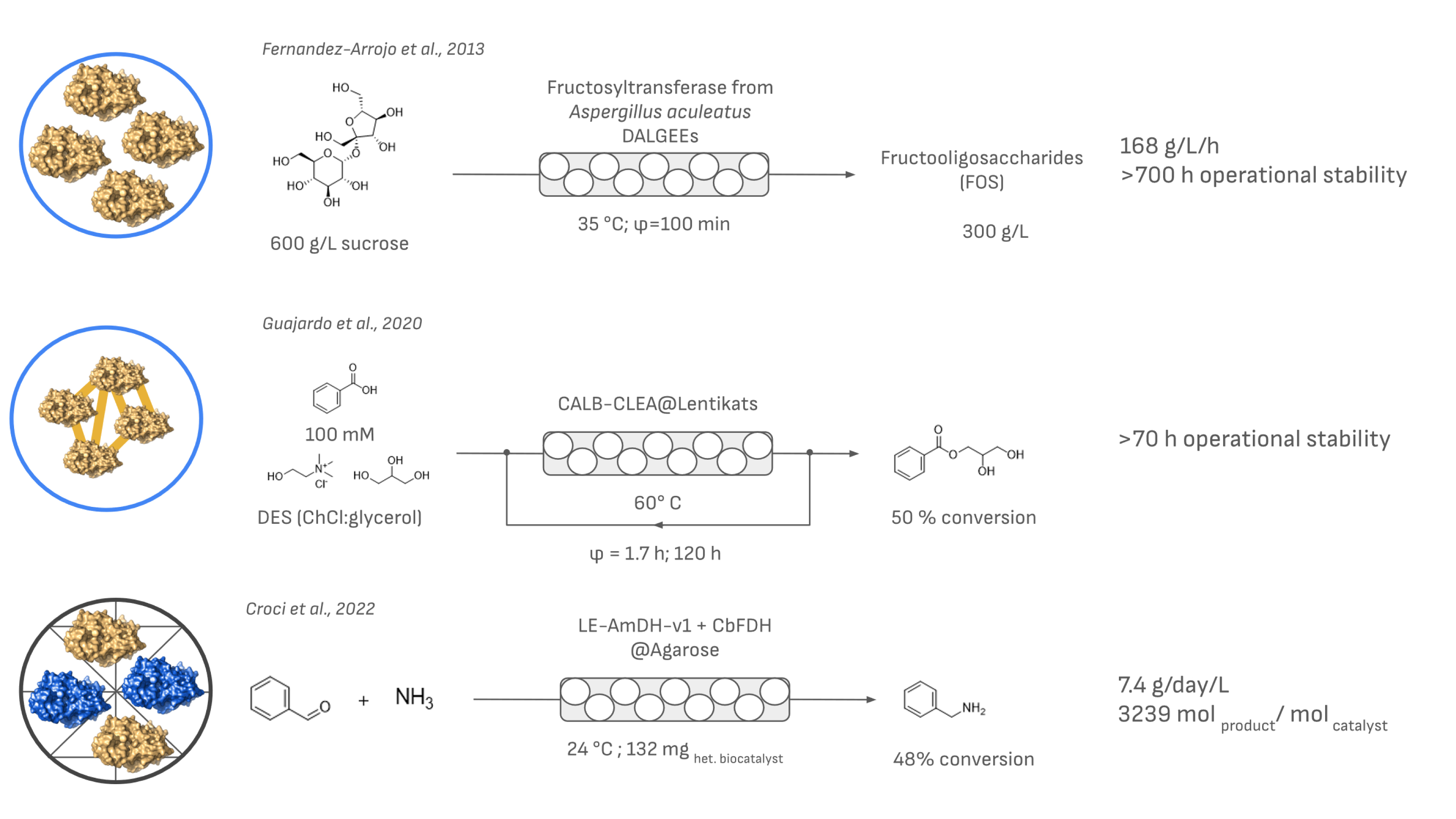
Selected examples of the use of entrapment and encapsulation methods in PBR systems. If specified in the original work, the contact time (φ) or the amount of biocatalyst used is indicated together with the space–time yield in g/L/h and either stability or productivity.
Another interesting option for encapsulation is Lentikats®. Reported 20 years ago, Lentikats® mediated encapsulation relies on the formation of polyvinyl acetate hydrogels under mild conditions, harnessing the excellent mechanical stability that this polymer offers. Interestingly, the cross-linking of the enzyme is essential for entrapment to be efficient in Lentikats® as discussed even in the first reports [48]. Several samples have been incorporated into PBRs (Figure 4). Guajardo et al. [49] used a combination of CLEA and Lentikats® entrapment to allow for the use of a lipase (CALB) in deep eutectic solvents, which served both as the reaction media and cosubstrate. First, by preparing CLEA–CALB followed by encapsulation in Lentikats®, they created a biocatalyst that could be incorporated into a PBR and exhibited higher reusability (>65%) after six cycles of 24 h at 60 °C when compared to CLEA–CALB alone.
More recently, with the rise of additive manufacturing (3D printing) for biocatalysis [50], Rabe et al. investigated the use of entrapped enzymes in a 3D printed agarose matrix and their application in PBRs [51]. In their initial work, a keto-isovalerate decarboxylase mutant with enhanced thermal stability was printed in disks created with agarose. The resulting materials were stacked within a column reactor and used in the continuous production of isobutyraldehyde. The resulting entrapped enzyme retained 36% of the activity and could be used for over 5 h continuously with minimal elution of the enzyme (0.7%). The same technology was used by Croci et al. [52], but this time for the co-immobilization of an amine dehydrogenase (LE-AmDH-v1) and a cofactor regeneration partner (CbFDH). In their study, the authors compared different immobilization techniques highlighting the necessity to tailor the immobilization process to the enzymatic system. Entrapment within the agarose matrices was, in their case, the best solution to avoid enzyme inactivation upon immobilization and to maintain the stability of the immobilized preparation. When used in flow, it achieved up to 7.4 g⋅L−1⋅day−1 of productivity and a total turnover of 3239 molproduct/molenzyme.
2.2.2. Carrier-bound immobilized enzymes for flow applications
Carrier-binding methods involve the interaction of enzymes with water-insoluble materials, such as natural polymers and inorganic materials. This binding can be through physical adsorption, or covalent bonding.
Physical adsorption
Physical adsorption is one of the simplest and the earliest methods used for enzyme immobilization. It involves the attachment of enzymes onto carriers through weak forces such as van der Waals forces, ionic interactions, and/or hydrogen bonding. This method typically does not alter the native structure of the enzyme, preserving most of its catalytic activity [53]. This method was first described in 1916 when Nelson and Griffin demonstrated that an invertase physically adsorbed onto charcoal remained catalytically active [28]. However, the weak nature of these interactions also means that it is easy for the enzyme to leach, detaching from the support even under gentle conditions, and, in most cases, does not significantly improve enzyme stability [54].
Despite the limitations, enzyme adsorption has been widely used in industrial processes and enabled continuous production [53, 55]. The most prominent example of this immobilization strategy is lipases [56], which have been extensively reviewed earlier [57, 58]. These enzymes are mostly used in biodiesel production and enantioselective transesterification or hydrolysis of esters and amides.
One example of adsorption immobilization in industrial flow biocatalysis is Lipozyme® TL IM from Novozymes, a lipase from Thermomyces lanuginosus (Figure 5). The enzyme is immobilized by ionic adsorption onto silicate supports. Lipozyme® TL IM can be used in non-aqueous environments as PBRs, as the hydrophilic carrier retains the necessary water to maintain enzyme activity even in the presence of oils or organic solvents. It has been successfully applied in many industrial processes [59], including inter-esterification of sesame oil and fully hydrogenated soybean oil at 70 °C in continuous-flow PBRs, demonstrating the practical applicability of adsorbed biocatalysts [60]. Other lipases immobilized with similar strategies have also been applied successfully. Of importance is Novozym 435, which has been applied to a myriad of esterification and hydrolysis reactions as a PBR [61, 62]. Another industrial use of adsorption immobilization is the production of allulose in continuous flow using ketose-3-epimerase immobilized on an ion exchange resin and integrated in a PBR [63, 64, 65]. This method has led to significant cost reductions and even the commercialization of DOLCIA PRIMA® Allulose, a low-calorie sweetener.
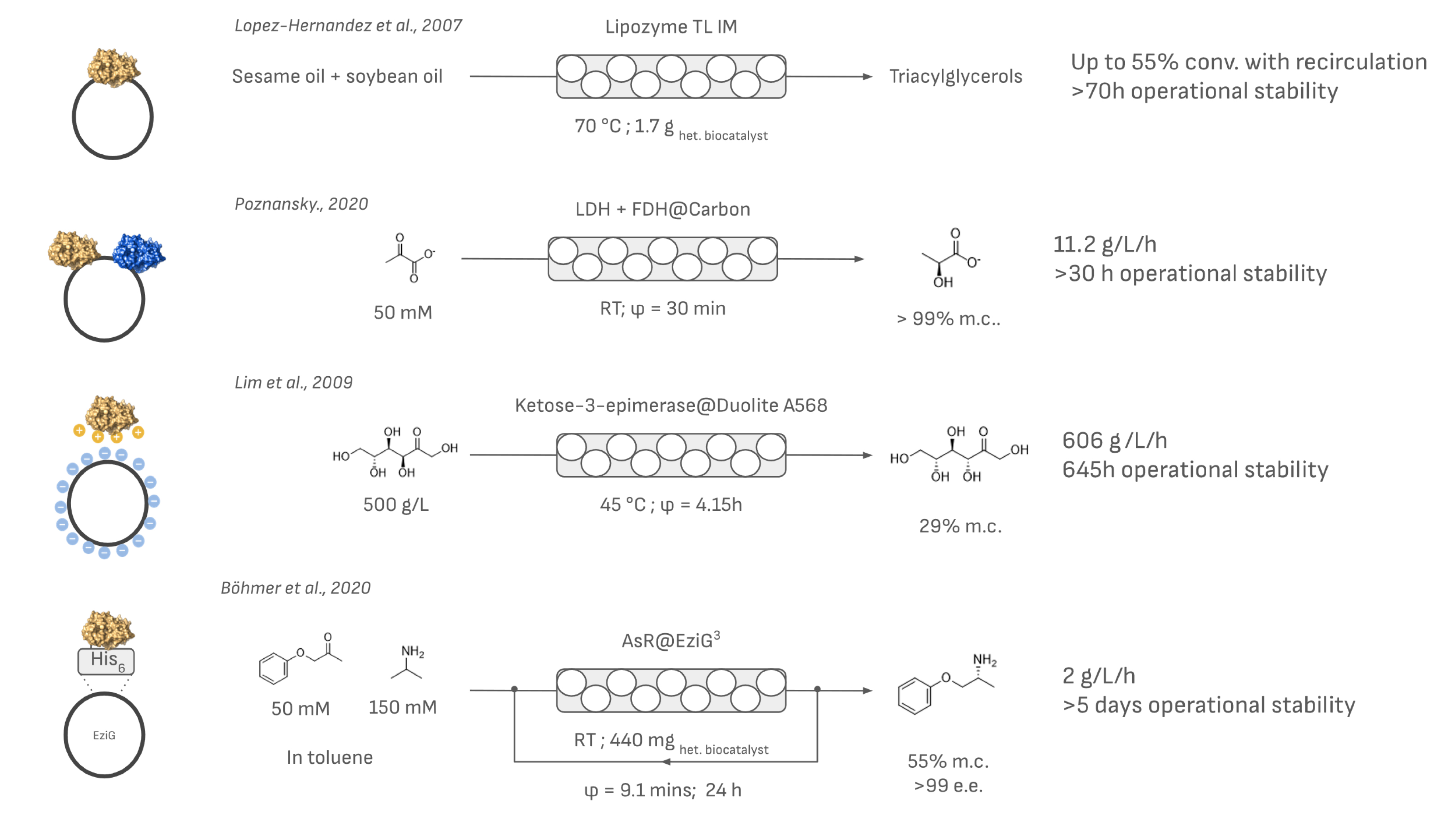
Examples of the application of non-covalently bound enzymes in a PBR system. If specified in the original work, the contact time (φ) or the amount of biocatalyst used is indicated together with the space–time yield in g/L/h and either stability or productivity.
Even multienzymatic systems have been immobilized using adsorption techniques (Figure 5). Co-immobilized lactate dehydrogenase and formate dehydrogenase on a carbon support facilitated ketone reduction with in situ cofactor recycling in a continuous-flow PBR. This system achieved a space–time yield of 11.2 g⋅L−1⋅h−1 in flow compared to 0.23 g⋅L−1⋅h−1 in batch processes, demonstrating the higher productivity achievable using low-flow reactor volumes (0.48 mL vs 24 mL in batch) [66].
Transaminases have also been immobilized through affinity adsorption using EziG™ materials. EziG™ is a hybrid controlled-pore glass coated with a functionalized polymer, engineered for selective metal-ion affinity immobilization of enzymes (Figure 5). It is designed for high loading of enzymes (up to 30% w/w). In a study performed by Böhmer et al., two stereoselective complementary ω-transaminases were immobilized onto EziG™, achieving 20% w/w loading. These immobilized enzymes were then utilized in a multigram-scale continuous-flow PBR for the production of (S)-α-MBA over 96 consecutive hours without any detectable loss of enzymatic activity [67, 68].
Covalent binding
Covalent immobilization involves the formation of stable covalent bonds between enzyme functional groups—such as amino, carboxyl, and hydroxyl groups—and those on a suitable carrier (Figure 6). This method has become important due to the strong linkages it forms through multipoint attachment, which significantly reduces enzyme leaching and enhances stability compared to non-covalent adsorption-based methods [69]. The use of covalent binding in enzyme immobilization has been reported in numerous reviews [3, 58, 70, 71].
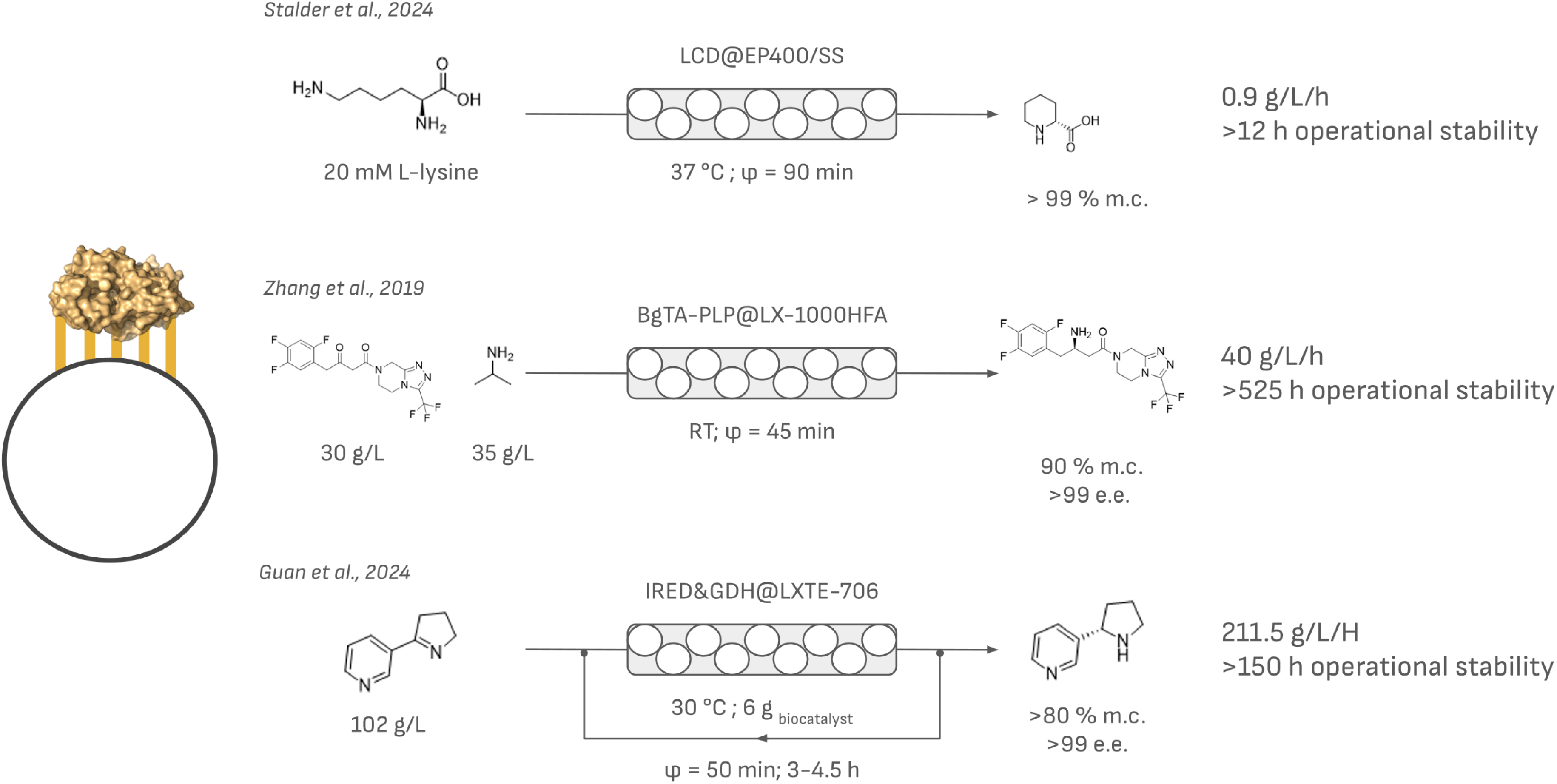
Selected examples of covalently bound enzymes applied in PBR. The contact time (φ) is indicated together with the space–time yield in g/L/h and either stability or productivity.
An exemplary case of covalent immobilization in flow biocatalysis is the enzymatic synthesis of L-pipecolic acid using covalently immobilized lysine cyclodeaminase [72]. This study compared the reaction between a SpinChem® reactor and a PBR. Batch reactions with the enzyme in solution resulted in low conversion yields and significant enzyme deactivation over time. Conversely, when employing continuous-flow reactors, full conversion was achieved within 90 min while maintaining high operational stability. Additionally, the study compared different immobilization methods, including physical adsorption and covalent binding, revealing that covalent immobilization provided better reusability by preventing enzyme leaching.
Another example is the biocatalytic synthesis of (R)-sitagliptin in a recirculating PBR. In this case, a transaminase was co-immobilized with PLP inspired by the creation of a self-sufficient biocatalyst by Benítez-Mateos et al. [73]. This biocatalyst maintained yields and enantiomeric excess values above 90% and 99%, respectively, over 700 batch reactions without the use of additional PLP. An overall space–time yield of 40.0 g/L/h was obtained [74].
Additionally, the synthesis of (S)-nornicotine was enhanced through the covalent co-immobilization of IRED and NADP-dependent GDH on an epoxy resin within a PBR. This system maintained substrate conversion rates above 99% after 114 h of uninterrupted operation and attained a space–time yield of 211.47 g/L/h, which is 289.7 times higher than that of batch processes [75].
3. Current trends and outlook
The integration of enzymes into PBRs is highly dependent on developing novel materials and immobilization techniques. Due to the complexity of these immobilization methods as discussed in this review, it is challenging to determine in advance which immobilized biocatalyst will perform best in flow systems.
Consequently, immobilization strategies are approached on a case-by-case basis. Ideally, the search should focus on strategies that can (1) minimize enzyme desorption and deactivation, (2) enhance substrate and product diffusion to maximize conversion, and (3) simplify downstream processing. However, with current techniques, there is always a balance between biocatalyst activity and stability. It is true though that stability is often favored when applying enzymes in PBRs. Although immobilized enzyme activity might be lower than free enzyme activity, the high local concentration of enzymes within the column allows for elevated substrate-to-catalyst ratios, resulting in higher conversion efficiency. Moreover, the precise control of reaction conditions within PBRs enables further process optimization. Adjustments such as increasing temperature, substrate loading, and implementing recirculation have been successfully employed to mitigate the lower activities observed in immobilized enzymes.
Innovation from material and chemical sides is still greatly needed in enzyme immobilization. Although immobilization has a long history, it remains predominantly based on interactions with charged amino acids, particularly the most reactive ones—almost exclusively cysteine and lysine or activated aspartic and glutamic acids. Therefore, developing new chemistry that can exploit other chemical handles is essential to ensure the process flexibility required for tailored immobilization strategies. Additionally, a deeper understanding of the interactions between enzymes and supports is important for optimizing these new immobilization techniques. Without this understanding, optimizing new immobilization strategies can be significantly hindered. Several key parameters of the support matrix are vital for maximizing the catalytic properties of immobilized enzymes. Consequently, investigations into novel formulations of bio-based polymers (such as lignocellulosic materials and innovative plastics), covalent- and metal-organic frameworks, and even solid materials previously regarded as waste are essential to enhance immobilization strategies [76, 77, 78].
Moreover, synergistically combining multiple immobilization techniques has shown promising results. Early examples included the combination of cross-linking and encapsulation. More recently, the integration of CLEAs with carrier-based immobilization has been employed to develop CLEANs (cross-linked enzyme-adhered nanoparticles), which have been applied to the synthesis of fragrances [79].
Adopting advanced screening technologies is crucial to identifying the most effective immobilization techniques. For example, López-Gallego and coworkers developed microtiter plate immobilization screening for high-throughput prototyping. This protocol tests 17 different carriers functionalized with various reactive groups in a 96-well plate format, simultaneously screening up to 21 immobilization protocols for up to 18 enzymes. By evaluating both activity and stability, this method enables the rapid identification of optimal immobilization chemistry, significantly accelerating the development of efficient multienzyme systems [80]. Integrating computational tools like CapiPy, a Python-based GUI application, further enhances this workflow by rationalizing screening results and facilitating informed decision-making for immobilization strategies [81].
Declaration of interests
Cristina Lía Fernández Regueiro and David Roura Padrosa are affiliated with inSEIT AG, and Francesca Paradisi is one of its founding members and its key scientific advisor.