1 Introduction
Artificial photosynthesis is one of the Holy Grails of modern science. The need for a clean and virtually infinite energy source, as solar energy is, in fact is becoming more and more urgent, when pollution arguments as well as the increasing and alarming rate at which our civilization consumes the fossil fuels are taken into account [1–4]. Moreover, the desired development of the parts of the World that at the moment do not benefit from the advantages of the so-called Western Countries will be made possible only if alternative energy resources are available.
A schematic representation of a potential artificial photosynthetic device is shown in Fig. 1. From the Fig. 1, it is clear that to perform this highly sophisticated function, many structurally-organized and functionally-integrated components are needed: (i) the antenna system, which has the function of collecting light energy and convey it to a specific subunit; (ii) the charge-separation component, which essentially should transform electronic energy into chemical (redox) energy; (iii) multielectron storage systems, which have to collect more than one electron (or hole) in reversible ways, also in successive steps, and deliver all the collected electrons (or holes) to suitable substrates in a single shot (most of the species which are potentially used to store chemical energy in usable forms need multielectron processes – this is the case for water splitting or carbon dioxide reduction, for example). It also could be noted that each component of this artificial photosynthetic device is indeed itself a multicomponent system. So, a hierarchical supramolecular organization is required.

Schematic representation of an artificial photosynthetic device.
The complexity of the system represented in Fig. 1 has for long time discouraged to face the problem in its completeness, which has been delayed until some crucial points were solved. In particular information on the rate constants of the various ultrafast processes involved and strategies to assemble the various components in efficient manners were required. However, the impressive developments of supramolecular chemistry on one side [5,6] and on ultrafast spectroscopy on the other side [7] during the last fifteen years opened the way to the design of multicomponent systems which have sound possibilities to explore the field. Of course, the straightforward approach to the design of efficient artificial photosynthetic systems is to face one component per time, i.e. to study the various components separately. Our interest was initially centred on the light-harvesting antenna system [8].
As Nature teaches, light-harvesting antennae have to be multichromophoric arrays in which the light energy absorbed by each active component (the single chromophores) is funnelled by a series of elementary energy transfer steps to a single, specific site [9–11]. So, two individual actions, light absorption and energy migration, are integrated to perform a multiple, more valuable function, the antenna effect. These considerations immediately indicate that synthetic strategies to build up in organized fashions as much chromophores as possible are needed. Dendrimers perfectly fulfil this requirement: their structure permits to concentrate in a limited space many subunits (in this case, they must be of course light-active subunits) by a limited number of synthetic steps. Furthermore, the divergent and convergent synthetic strategies allow us to insert modifications in the various 'layers', and so energy gradients can be synthetically predetermined.
Here we illustrate in some detail the synthetic strategy adopted by us to prepare a large number of light-harvesting dendrimers made of Ru(II) and Os(II) polypyridine building blocks, also in collaboration with colleagues in Bologna and Pisa [8, 12–26], and report on the properties of some selected examples of this class of compounds.
2 The building blocks
If an artificial antenna system has to be developed, the obvious choice is to think of it as a modular system, as well as Nature did. This leads us to focus directly on the individual modules to be used (the building blocks). The requirements that the building blocks have to fulfil are the following: (i) stability in the ground and excited states; (ii) capability to significantly absorb solar light; (iii) relatively long-lived excited state, to allow the occurring of energy transfer with minimal loss of energy. In particular, point (i) is even more important for artificial antennas than for natural ones, in that self-repairing processes, available in Nature, are difficult to reproduce in artificial systems. We have employed as building blocks Ru(II) and Os(II) polypyridine subunits. Primarily this is because these species exhibit an unusual combination of properties rarely found simultaneously in other compounds [27, 28]. The relevant properties of Ru(II) and Os(II) polypyridine complexes are indeed: (i) good stability of the ground as well as the excited and redox states; (ii) absorption in the visible region, due to intense spin-allowed (and in the case of osmium compounds, also spin-forbidden) metal-to-ligand charge transfer (MLCT) bands; (iii) relatively long-lived (typically in the microsecond time range) and luminescent excited states. Emission is usually due to radiative deactivation of the lowest-lying 3MLCT level(s); (iv) reversible metal-centred oxidation and ligand-centred reduction processes at accessible potentials; (v) tunability of all the properties by a judicious choice and combination of the ligands.
It also should be considered that the contemporary presence of several Ru(II) and/or Os(II) polypyridine complexes within the same polynuclear structure adds new properties to the multimetal arrays, due to the supramolecular nature of these systems [29, 30] besides the basic properties of the building blocks cited above. The new properties depend on the electronic interactions between the various subunits, which is in turn due to the connections used and the topography of the systems. Among the various (supramolecular) properties that Ru(II)- and Os(II)-based polynuclear complexes can exhibit, photo-induced energy transfer processes within the arrays and simultaneous multielectron redox processes are particularly intriguing here since they can lead to useful functions, such as light-harvesting antenna effect for solar energy conversion purposes and multielectron/hole storage [8, 31, 32].
The components used to build up the dendrimers described in this article are shown in Fig. 2, together with their schematic representation. Some dendrimers of different shapes are shown in Fig. 3.

Components of the dendrimers, and their schematic representation.
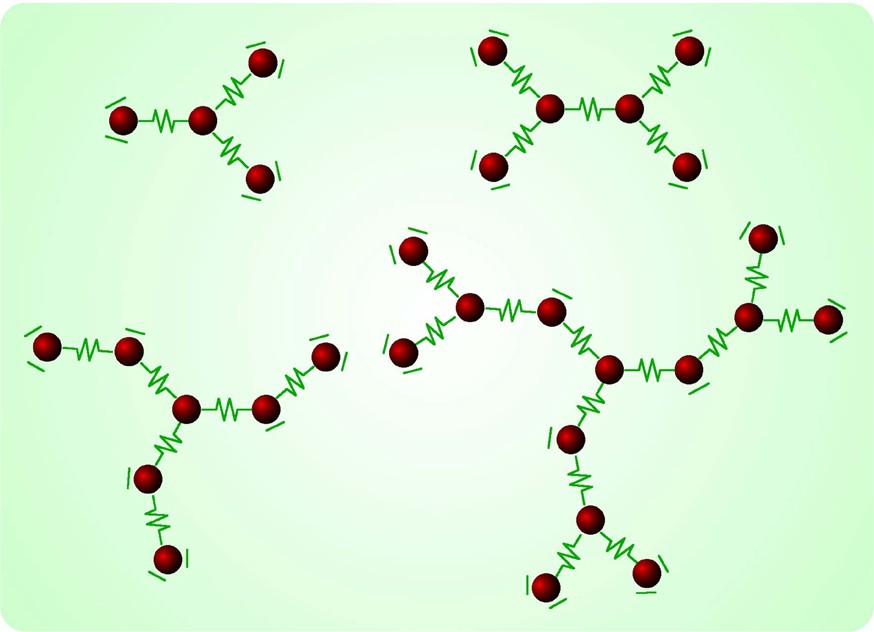
Schematic representation of some dendrimers obtained in our laboratories (details can be found in the original references [12–15]). All these species, as well as the other mentioned in this article, are highly charged cations (each metal subunit introduces two positive charges) and have been prepared as hexafluorophosphate salts.
3 Synthetic strategy: the ‘Complexes as ligands and complexes as metals’ approach
The synthetic strategy used to build up the metallodendrimers here described is an example of modular synthetic chemistry [5, 33], a general approach that is becoming more and more adopted in chemical synthesis and has its roots in supramolecular chemistry. The basic concept is that to obtain large (supra)molecular edifices, it is convenient to prepare simpler multicomponent structures (modules) and to assemble them into structurally organized systems by taking advantage of some specific complementary reaction sites.
Mononuclear transition metal complexes are typically synthesized by reacting metal ions (M) and free ligands (L) as shown in Eq. (1).
The ‘complexes as ligands and complexes as metals’ approach is based on the use of complexes instead of simple metal ions and free ligands [22, 34]. The place of M in Eq. (1) is therefore taken by mono- or oligonuclear complexes which possess ligands that can be readily substituted, so that species with unsaturated metal coordination sites can be easily generated under the reaction conditions employed (‘complex metals’), and the place of L can be taken by mono or oligonuclear complexes which contain free chelating sites (‘complex ligands’).
The ‘cal/cam’ approach shows its full potential when polynuclear and/or multifunctional complex ligands and complex metals are available. This is exemplified in Fig. 4, where a trifunctional mononuclear complex ligand (containing free chelating sites) and a monofunctional, trinuclear complex metal are reacted to form a decanuclear complex. The reaction shown in Fig. 4 warrants some comment. (i) A complex of high nuclearity and dendritic shape is prepared in a single step by taking advantage of the complementary functionalities of the reaction partners. (ii) The topography of the final structure is fully controlled, in the sense that: the metal centre in the central position (Mc) of the decanuclear species is the metal originally belonging to the trifunctional complex ligand; the metal centres in the intermediate positions (Mi) are the metals of the trinuclear complex metal carrying the leaving chloro ligands; and the metals in the peripheral positions (Mp) are the peripheral metals of the parent trinuclear complex metal. An analogous control on the position of the bridging ligands can also be obtained. (iii) The decanuclear species is a second-generation dendrimer, obtained by a convergent synthesis. It should be considered that point (ii) in particular is made possible by the stability of the Ru– and Os-polypyridine complexes. Indeed, a prerequisite for the application of this method is that ligand scrambling does not take place.
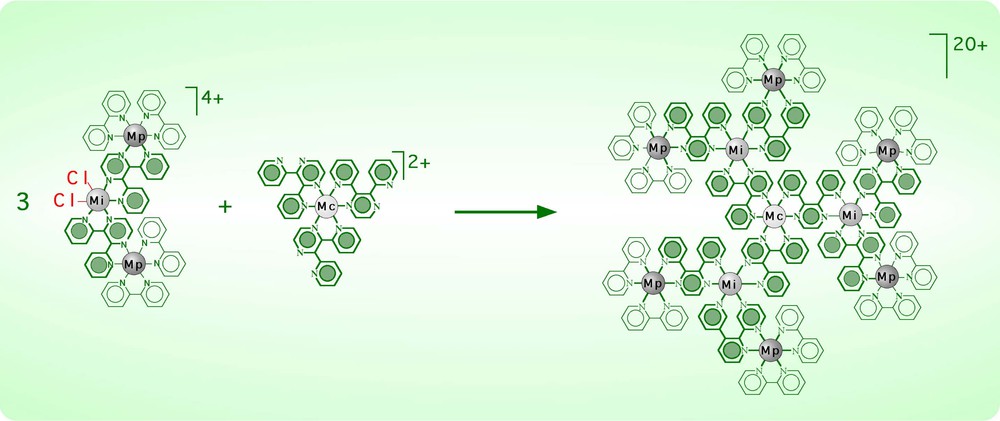
Synthesis of a decanuclear complex by the ‘cal/cam’ approach (Mc = central metal; Mi = intermediate metal; Mp = peripheral metal).
Decanuclear complexes such as the one showed in Fig. 4 lead us into the dendrimer realm. Dendrimers are usually prepared by two approaches [35–38]: one involves a divergent iterative coupling and activation protocol from the core (divergent approach), whilst the other is based on the preparation of dendrimer branches (dendrons) and the connection of the various branches to a single focal point, i.e. the core (convergent approach). However, alternative strategies have also been reported [35, 39–41]. The synthesis of decanuclear complexes schematised in Fig. 4 is an example of the convergent approach. As mentioned above, decanuclear complexes of the type discussed here are second-generation dendrimers, in which the complex ligand [Mc(dpp)3]2+ plays the role of the core in the final structure, with each one of the three-trinuclear complex metals acting as dendrons.
Several decanuclear dendrimers containing Ru(II) and Os(II) subunits have been prepared by this approach, containing different metals in the various ‘shells’ and different peripheral ligands, thanks to the availability of different complex metal dendrons and complex ligand cores [22]. Different schematic representations of one of these dendrimers, namely [Ru{(μ-2,3–dpp)Ru[(μ-2,3–dpp)Ru(bpy)2]2}2]20+, are shown in Fig. 5, in which the three-dimensional nature of these species is apparent.
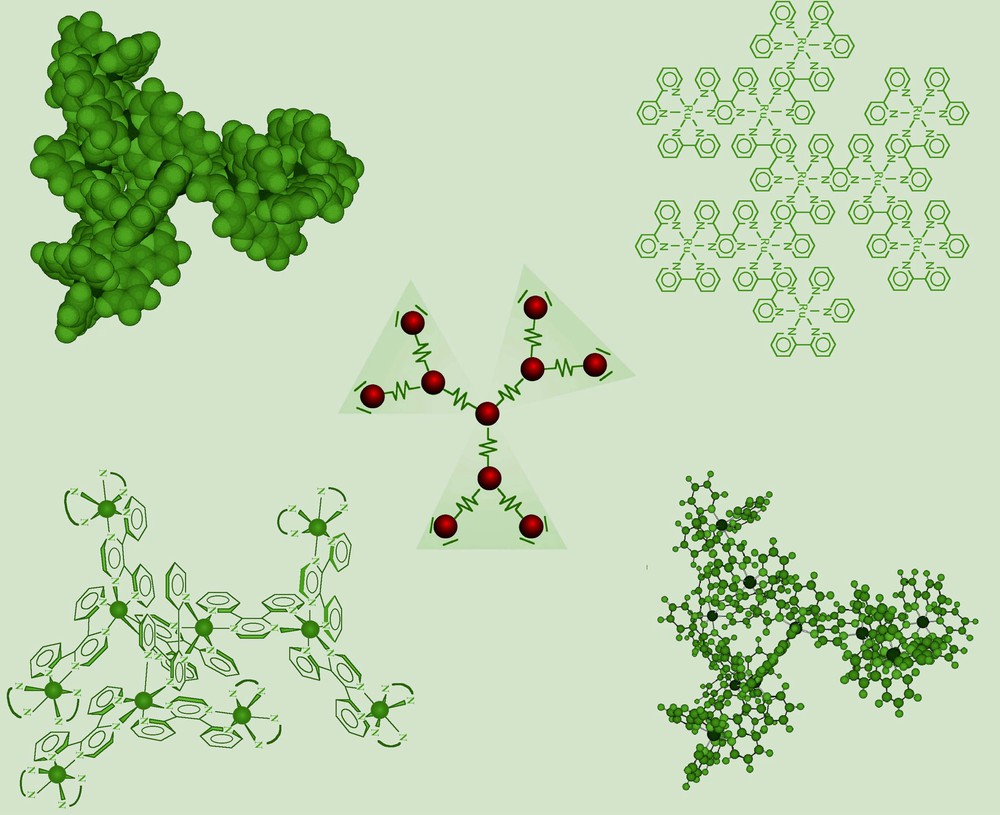
Different representations of a decanuclear dendrimer. Charges are omitted for clarity.
An important breakthrough in the field of metal-dendrimer synthesis came from the implementation of an iterative process within the ‘cal/cam’ synthetic framework, based on a protection/deprotection protocol of polypyridine chelating sites [18, 34]. Indeed, all the divergent syntheses of dendrimers rely upon an iterative coupling of subunits and require the availability of a species carrying two types of functional groups. In the ‘cal/cam’ approach, this means the availability of a species that can behave both as a complex ligand and as a complex metal. A simple example is the compound Ru(2,3–dpp)2Cl2 (Fig. 6). However, a species like this is self-reactive under the preparative conditions because the free chelating site of one molecule could substitute the labile chlorides of another molecule, leading to an uncontrolled mixture of species. The key step towards the preparation of the required compound was the synthesis of the monomethylated 2,3–Medpp+ ligand (Fig. 2), which allowed the preparation of the [Cl2Ru(2,3–Medpp)2]2+ complex (Fig. 6) [18]. This latter complex is a masked bifunctional compound, in that it is a complex metal but also a potential complex ligand: its potential as a complex ligand can be activated by demethylation. Reacting the masked bifunctional complex [Cl2Ru(2,3–Medpp)2]2+ with [Mc(2,3–dpp)3]2+ cores affords tetranuclear-protected compounds (first generation dendrimers). After deprotection, the reaction can be iterated. The general procedure is shown in Fig. 7. Two essential steps can be distinguished here, the deprotection and the growth reactions. The deprotection reaction leads to different dendrimers of the same generation, while successive generations are obtained by the growth reaction. It is also interesting to note that each deprotected dendrimer can be used as a core in convergent synthetic processes. This strategy was employed in the syntheses of the two largest dendrimers of this series obtained so far, the docosanuclear species (i.e. containing 22 metal centres) schematically shown in Fig. 8 [18,20]. Both compounds were prepared by reacting the appropriate tetranuclear deprotected species (i.e. hexafunctional complex ligands, carrying six free chelating sites at the periphery) with six trinuclear complexes of the type shown in Fig. 4 (a multifunctional complex metal species).

Example of Ru(II) complex used in the ‘cal/cam’ approach (unprotected and protected forms).

General procedure of the divergent approach. Each deprotected dendrimer can be used as a core in a convergent approach.

Docosanuclear species. Charges are omitted for clarity.
4 Ru(II) and Os(II) dendrimers as light-harvesting antennae. General properties and selected examples
For light-harvesting antenna systems, relevant aspects are: cross section with solar light output (i.e., absorption of sun light) and efficiency of energy migration within the multichromophoric array to convey the absorbed electronic energy to a specific site (i.e., directional energy transfer). Absorption of sunlight in the dendrimers described here is accomplished by the contemporary actions of all the metal chromophores of the arrays. Actually, each metal-based unit exhibits intense ligand centred (LC) bands in the UV region and moderately intense metal-to-ligand charge transfer (MLCT) bands in the visible. As it is shown by the electrochemical behaviour [32, 42], in the dendritic (polynuclear) species there is some interaction among the neighbouring metal-based units. To a first approximation, however, each building block carries its own absorption properties in the polynuclear species, so that the molar absorption coefficients exhibited by the compounds increase with the nuclearity of dendrimers. To exemplify this concept, Fig. 9 shows the absorption spectra of three dendrimers of different generations. As it can be seen, the molar absorption coefficient of the docosanuclear species reaches the huge value of 200 000 M–1 cm–1 at 545 nm, remaining also significantly high in most regions of the visible spectrum.

Absorption spectra of three dendrimers of successive generations.
As far as directional energy transfer is concerned, luminescence experiments have shown that energy transfer between nearby subunits is practically quantitative and ultrafast when the process is exoergonic or even when the relevant excited states are isoergonic [8]. This is shown by the schematisation in Fig. 10. For example, in the Os-containing tetranuclear dendrimer, the electronic energy collected by the peripheral Ru-based subunits is transferred quantitatively to the Os-based core, which behaves as the energy trap of the antenna (Fig. 10, case a). However, energy migration becomes inefficient between units that are separated by components with higher-lying excited states (Fig. 10, cases b and c). This apparently limits the performance of some larger dendrimers as antennae. However, some recent investigations might demonstrate that this limitation can be overcome for many potential applications (see later).

Schematisation of the energy migration processes occurring in some dendrimers.
5 Recent developments and future directions
For several years the study of the photo-induced energy transfer processes within the metal dendrimers here discussed was limited to steady-state experiments, and the rate constants of the various processes remained untouched. This because the processes take place on a timescale (subnanoseconds) that was not accessible by usual techniques. Many questions concerning the mechanisms of such ultrafast processes remained therefore unsolved. Very recently, we started to re-investigate these processes by ultrafast (femtosecond) spectroscopy [43, 44]. This allowed us to gain key pieces of information which shine light on some topics concerning the properties of these class of metallodendrimers, help to rationalize some particular behaviour and, more importantly, suggest future developments. These new very recent findings can be grouped into two subtopics: (i) the possibility of the involvement of non-equilibrated states in the energy transfer processes and (ii) the possibility of long-range electron transfer processes that can also mediate energy transfer between distant subunits.
5.1 Energy transfer from non-equilibrated excited states
Recent experiments on dinuclear [43] and tetranuclear [44] species of this class of compounds clearly indicate that energy transfer between nearby units can be faster than 200 fs. On considering that intersystem crossing as well as other relaxation processes within each excited subunit occurs in the same timescale, it can be inferred that singlet-singlet energy transfer can play an important role in the overall process. The fundamental consequence is that the general approach to discuss energy transfer processes between metal polypyridine complexes, which is based on triplet-triplet energy transfer, probably must be reconsidered, at least for cases where electronic coupling between the partners is not negligible, as it is the case for the species here presented (i.e., when redox properties indicate that interaction between the metal subunits takes place). New avenues for investigation are opened, which can also have important consequences on the applicative point of view. For example, the above described findings are important for the construction of larger antennas, photonic wires, and other molecular devices where a rapid and efficient energy transfer is desired over a large distance. On the basis of our results, a very rapid energy transfer in each step can compete efficiently with other excited state deactivation pathways, and result in small losses of excitation also in multi-step systems. In the present dendrimers, the lowest 3MLCT state of any subunit has an intrinsic lifetime of ca. 100 ns [8]. Since energy transfer between nearby subunits is at least 5 × 105 times faster, this suggests that the probability of excitation loss is only 2 × 10–6 in each step. This consideration perfectly fits with the quantitative energy transfer measured for large dendrimers as the one in Fig. 10, case d. Much larger dendrimers of the same type can therefore be designed, which are still expected to exhibit quite high-energy migration efficiencies.
5.2 Long-range electron transfer
The occurring of long-range electron transfer has been recently proved for a system that couples a trinuclear dendron with an electron donor subunit [45]. The system, which represents the first example of integrated antenna/charge separation components involving metal chromophores, is shown in Fig. 11. In this species, the lowest excited state of the trinuclear dendron is centred in one of the peripheral Ru-based subunits, which are separated from the tetrathiafulvalene electron donor component (TTF) by another metal chromophore having a higher-energy excited state. However, electron transfer from TTF to the peripheral excited subunit(s) takes place in 300 ps. Preliminary results on a similar species (involving a heptanuclear, second generation dendron as the antenna component) qualitatively confirm this finding [46]. The result can have useful consequence both on the design of efficient artificial photosynthetic systems and on the possibility of interfacing such type of dendrimers with suitable surfaces with a good hope for obtaining useful functions (if this effect will be confirmed, it would imply that could not be essential to connect the charge-separation devices or the surface elements with the energy trap of the dendrimer to obtain some required properties). Finally, recent results also demonstrate that energy transfer between distant subunits can be efficiently mediated by long-range electron transfer steps [47], which probably is a special case of the efficiency of electron transfer on long distance operating in these metal dendrimers.

TTF-containing trinuclear dendrimer.
On the basis of the above mentioned very recent results, many properties can probably be reconducted to a few general concepts (ultrafast energy transfer between nearby subunits and long-range electron transfer) which could explain in full detail the behaviour of these metal-based dendrimers, also opening new and unexpected perspectives, which can also assume some relevance in related areas of research. Work is in progress in our laboratories towards these directions.
Finally, we would like to make in evidence a funny event (absolutely non scientific): the word ‘dendrimers’ was derived from the Greek word meaning 'tree'. Trees (i.e., green plants) are the most effective (natural) photosynthetic systems. The use of dendrimers as light-harvesting antenna systems for artificial photosynthesis almost appears as a sort of nemesis for this class of fascinating new systems.
Acknowledgements
S.C. and S.S. wish to express their gratitude to Prof. Vincenzo Balzani for his fundamental teaching, for having stimulated their interest in photochemistry and supramolecular chemistry and for continuous support and friendship. The European Community (TMR Network on Nanometer size metal complexes, contract n. ERBFMRX-CT-98-0226) and MIUR are acknowledged for financial contribution. F.L. thanks a Marie Curie fellowship grant.