1 Introduction
Solid-state NMR spectroscopy has emerged as a powerful tool to characterise the structure and dynamics of membrane proteins by using static oriented samples or magic angle sample spinning techniques [1–3]. Proteins can be studied when associated with hydrated liquid crystalline bilayers, where a high degree of lateral and rotational diffusion is present. To perform solid-state NMR on static samples, proteins are reconstituted into oriented bilayers and the samples are introduced into the magnet with their normal parallel to the magnetic field. Alternatively, bicelles provide model membranes, which orient spontaneously in the magnetic field [4,5]. Partially averaged anisotropic magnetic interactions (chemical shift anisotropies, dipolar and quadrupolar couplings) provide local probes of protein dynamics and orientation [2,3,6,7] and of protein–lipid interactions [8,9].
OmpA is one of the major proteins in the outer membrane of gram-negative bacteria (present at about 105 copies per cell) and is highly conserved among this family. Its N-terminal transmembrane domain is a prototype of β-barrel proteins whose three dimensional structure has been solved by X-ray crystallography [10] and by NMR [11,12]. As such it is an interesting model to understand β-barrel membrane protein dynamics [13], which is complementary to purely α-helical proteins such as bacteriorhodopsin [14]. Klebsiella pneumoniae OmpA transmembrane domain (named hereafter KpOmpA) shows a high degree of sequence identity with the well-known E. coli OmpA, and presents a pharmacological interest for its adjuvant property and carrier protein activity [15,16].
In order to analyse KpOmpA dynamics by solid-state NMR we had to prepare oriented bilayers with a mosaic spread as low as possible. Preliminary attempts, using a standard protocol based on the fusion of small unilamellar vesicles (SUVs) onto glass plates by several cycles of dehydration–rehydration [8], failed to provide high-quality orientation. Another difficulty often encountered when using proteo-liposomes formed from detergent solutions of the membrane protein is that many preparation give rise to large unilamellar vesicles with sizes ranging from 100 to 500 nm. These vesicles are not suitable for 2H NMR analyses because the lipid reorientation is not sufficiently slow on 2H NMR time scale. It is, however, important to compare powder spectra with oriented bilayers spectra, to make sure that the oriented bilayer stacks are sufficiently hydrated and have properties identical to vesicles in excess water. In this paper we used the protocol recently published to prepare giant protein containing vesicles (GVs) by electroformation [17], and found that GVs are not only suitable to observe standard 2H NMR powder spectra, but are also excellent intermediates in the preparation of oriented bilayers containing a high protein to lipid ratio.
2 Materials and methods
cDNA cloning, expression, purification and refolding of KpOmpA: KpOmpA protein (Swiss-Prot entry name OMPA_KLEPN, primary accession number P24017) was produced and purified according to [15], with the following modifications. From the plasmid pVALP40, kindly provided by the CIPF, Pierre Fabre, the Xba I–HindIII DNA fragment, corresponding to the membrane domain (Met 1–Glu 207) was introduced in the linear NheI–HindIII expression vector pET21c. Escherichia coli BL21DE3 were used for the expression of the His-tag fusion KpOmpA membrane domain. Being produced in inclusion bodies, the fusion protein was first solubilised in 7 M urea and refolded in Tris buffer containing 1% Zwittergent 3–14. The purification was performed on a Ni2+–IDA resin using a standard procedure. The resulting 216 aa protein contains six histines at its C-terminus and three amino acids (Ala–Arg–Ile) before the first Met of KpOmpA.
Multilayered vesicles (MLVs) of the mixture DMPCd54 (Avanti Polar Lipids (Alabaster, AL, USA) and DLPC (Genzyme Pharmaceuticals, Liestal, Switzerland) were prepared from 5.3 mg of dry DMPCd54/DLPC (25:75, mol/mol) dispersed in 440 μl of deuterium depleted water (Cambridge Isotope Laboratories, Andover, USA). The MLVs could be analysed directly by 2H NMR or sonicated using a tip sonifier (Branson Model 250 sonifier, 15 min, 50% duty cycle, power 30 W), in order to obtain SUVs for protein reconstitution experiments.
KpOmpA was incorporated into proteoliposomes according to the general procedure developed by Rigaud and co-workers [18]. Aliquots of zwittergent 3–14 (Calbiochem, CA) solubilised proteins were added to the detergent-lipid mixture under stirring at the desired protein to lipid molar ratio. Reconstitution of KpOmpA was obtained by removing the detergent with pre-washed SM2 Bio-Beads (Biorad, CA) [19]. KpOmpA incorporation and folding state into DMPCd54/DLPC bilayers were checked by means of linear sucrose density gradient centrifugation and 8% polyacrylamide gel SDS-PAGE. For 2H NMR investigations KpOmpA/DMPCd54-DLPC (1:50 mol/mol) giant vesicles were prepared.
Giant vesicles (GVs) were generated by the electroformation technique [17,20]. Preformed liposomes or proteoliposomes were carefully deposited, by droplets on indium tin oxide (ITO) covered glass slides (8 mg lipids on 4 × 1 cm2). The film was then partially dried 30 min under vacuum. The two ITO slides were sealed with a 1-mm spacer. About 400 μl H2O depleted D2O was added and the conductive glasses were connected to the generator with a conductive silver resin (Ecolit 340, Eleco produits, Fr). For electroformation, an AC electric field provided by a square-wave pulse generator was applied at room temperature across the chamber. The voltage was incremented first every 6 min from 65 mV to 1 V at 8-Hz frequency and then kept for 12 h at 1 V. Then, the AC frequency was lowered to 4 Hz, and the voltage was raised to 1.5 V for 2 h to detach the giant vesicles from the glass slides. Giant vesicles morphology was checked by phase contrast optical microscopy. Twenty microlitres of the giant vesicles suspension diluted to 3 mg of lipids/ml were deposited in a Labteck II chambered coverglass system (Nunc, Naperville, IL). The chamber was placed on the stage of an inverted digitised microscope (Leica DMIRB, Wetzlar, Germany) 30 min before the experiment, to allow GV sedimentation. Giant vesicles were observed and images were recorded with the CELLscan System from Scanalytics (Billerica, MA) equipped with a Photometrics cooled CCD camera (12 bit grey levels) (Princeton Instrument, Inc.).
Oriented samples of DMPCd54/DLPC (25:75 mol/mol) and KpOmpA/DMPCd54-DLPC at a protein to lipid molar ratio of 1/50 were prepared from giant vesicles suspensions diluted to a lipid concentration of 12.5 mg/ml in deuterium-depleted water. The giant vesicles were then gently sonicated in a ‘bath’ sonifier (this procedure was shown to give a more uniform size distribution around 1–5 μm by breaking larger vesicles, and to improve the quality of orientation) and uniformly spread on 15 glass plates (5 × 10 mm2, 40 μl per plate), previously pickled overnight in fuming nitric acid, rinsed carefully with water and dried. After solvent evaporation under vacuum, five cycles of dehydration–rehydration (in a water-saturated atmosphere, 40 °C) were applied to the sample. The sample surface density was calculated to be 1 mg per cm2. Before NMR experiment, the hydrated plates were stacked in a KelF tube (7-mm outer diameter, 25-mm long) and the sample was equilibrated 30 min at 30 °C. 40 μl of deuterium depleted water was added inside the NMR tube cap in order to ensure permanent excess hydration of the sample. The measured sample weight was 7.5 mg (3.1 mg of protein and 4.4 mg of lipid mixture).
Solid-state 2H NMR experiments were carried out on a Bruker DMX narrow bore spectrometer operating at 500.17 MHz for 1H. A Bruker static single-tune 5-mm solenoid-coil was used for liposomes suspension analysis whereas a double-tuned 7-mm solenoid-coil was used for oriented samples. Both solenoid coils were oriented at 90° with respect to the magnetic field. 2H RMN spectra were recorded at 76.77 MHz using a standard quadrupolar echo pulse sequence [90x-t-90y-t-acq] [21] (4.0 μs 90° pulse on 5-mm solenoid coil, 5 μs 90° pulse on 7-mm solenoid coil, 40 μs interpulse delay, 4.2 ms acquisition time, 1 s recycle delay, and 500 kHz spectral width). Typically, 12 000 scans were accumulated. All spectra were recorded at 30 °C, i.e. above the phase transition temperature of the phospholipid mixture (shown to be equal to 6 °C).
3 Results and discussion
3.1 DMPCd54/DLPC bilayers
Fig. 1a, b and c show 2H NMR spectra recorded at 30 °C of large unilamellar vesicles (LUVs, obtained after filtration of MLVs on 0.4-μm polycarbonate filters), multilamellar vesicles (MLVs) and giant vesicle suspensions (GVs), respectively. The 2H NMR spectral line shape of MLVs (powder spectrum, Fig. 1b) consists of the motionally-narrowed Pake-doublet powder pattern. This line shape is typical of axially symmetric movements. This symmetry arises from the fast axial diffusion of the phospholipids in a liquid crystalline bilayer and results in a rapid anisotropic motional averaging about their long molecular axis that is parallel to the normal bilayer. This axis of diffusion has been confirmed by examination of the angular dependence of quadrupolar splittings as a function of the bilayer orientation (Fig. 1d). Compared to this reference spectrum, the spectrum obtained with LUVs (Fig. 1a) clearly shows intense deformations. These are due to the insufficient vesicle size, which leads to further averaging of 2H quadruple splittings through vesicle tumbling and/or lateral diffusion within the bilayer plane. Smaller vesicles (SUVs) would finally give one sharp line (isotropic diffusion limit). Compared to this spectrum, GVs (Fig. 1c) give a spectrum very similar to the reference spectrum of MLVs. Measurements of plateau and methyl 2H quadrupolar splittings of DMPCd54 in giant vesicles suspension were compared to those measured in MLVs (values were collected in Table 1 with an experimental error evaluated on four independent experiments at ±500 Hz and ±100 Hz for plateau and methyl 2H quadrupolar splittings, respectively). 2H quadrupolar splittings are constant in both samples. Therefore, there are no significant isotropic motional averaging effects from overall vesicle tumbling and lipid lateral diffusion within the bilayer plane in GVs sample. As shown by phase contrast image presented in Fig. 2, GVs generated by the electroformation technique display a broad size distribution with diameters varying between 1 and 100 μm in agreement with the literature [22]. In such sample, the correlation times for vesicle tumbling and reorientation via lipid lateral diffusion are slower than the 2H NMR time scale (10−5 s) and, therefore, display no deleterious vesicle size effects on NMR spectra. Furthermore, we have determined that neither the phase transition temperature (6 ± 1 °C), nor T2e relaxation parameter of hydrocarbon chains of DMPCd54 were affected (data not shown). Nevertheless, a reduction in apparent linewidth and a better resolution were observed in giant vesicle sample as compared with MLV suspension. The comparison of Fig. 1b and Fig. 1c clearly shows a reduced intensity at 5–10 kHz and a better resolution of the peaks for the giant vesicle sample. This can be accounted for by a deformation of the spherical vesicles into ellipsoid with a larger proportion of bilayer planes oriented parallel to the magnetic field. Indeed, it is known that, due to their negative magnetic susceptibility anisotropy, phospholipids bilayer planes, like phospholipid bicelles, tend to orient themselves parallel to the magnetic field [4].

2H NMR spectra of bilayers composed of 2H-labelled DMPCd54/DLPC (25:75 mol/mol), 303 K. a) suspension of LUVs (0.4 μm diameter), b) suspension of MLVs, c) suspension of GVs, d) Oriented bilayers, bilayer normal aligned with magnetic field (0° OB).
2H quadruple splittings of methylene plateau (CH2) and methyl (CH3) resonance of DMPCd54/DLPC (25:75 mol:mol) bilayers in multi lamellar vesicles (MLVs), giant vesicles (GVs) and 0 degree oriented bilayers (0° OB); without KpOmpA (–) and with KpOmpA at a protein to lipid ratio of 1:50 (+)
Experimental errors on methylene plateau and methyl are estimated at ±500 Hz and ±100 Hz, respectively
KpOmpA | CH2 (Hz) | CH3 (Hz) | |
MLV DMPCd54/DLPC | – | 26 730 | 2840 |
GV DMPCd54/DLPC | – | 26 700 | 3000 |
+ | 26 200 | 2880 | |
0° OB DMPCd54/DLPC | – | 52 160 | 5800 |
+ | 52 000 | 5930 |
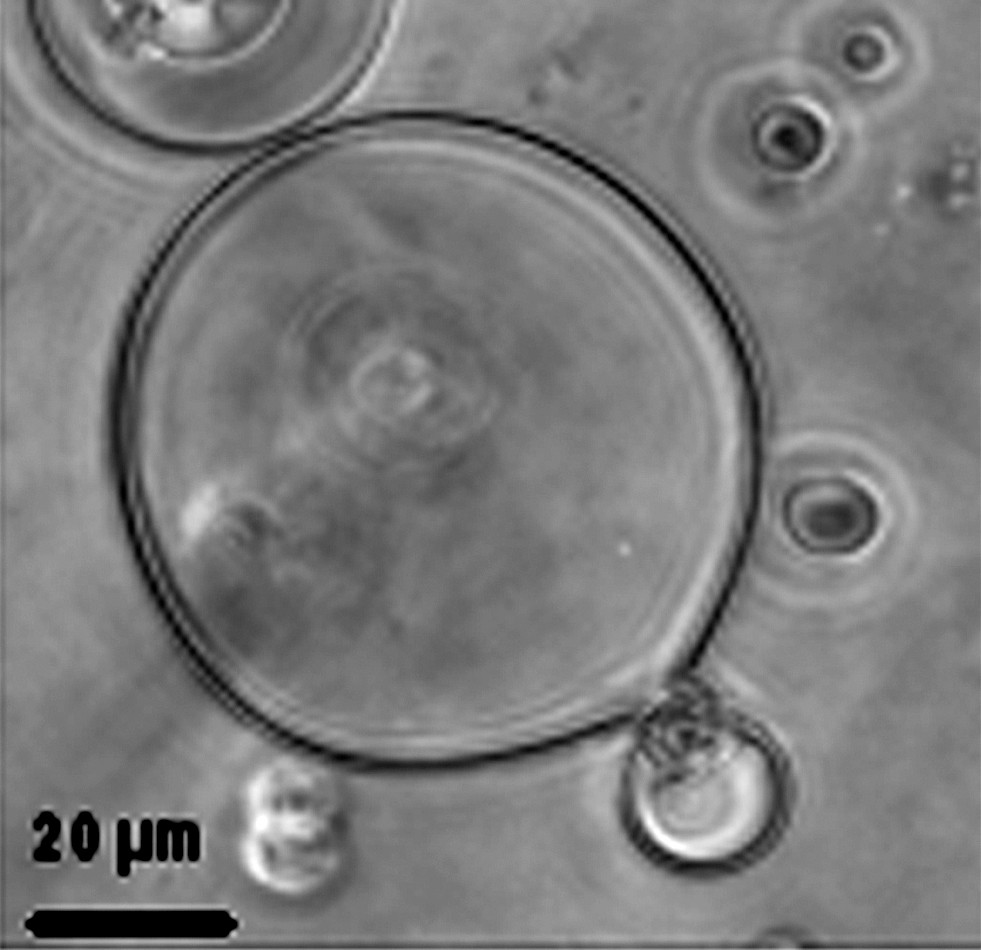
Phase contrast image of pure DMPCd54-DLPC (25:75 mol/mol) giant vesicles formed by the electroformation technique.
Oriented membranes were prepared from DMPCd54/DLPC (25:75 mol/mol) GVs suspension in order to study the dynamic behaviour of DMPCd54 with a better resolution. As shown in Fig. 1d, 2H NMR of bilayers oriented with their normal parallel to the magnetic field shows a well-oriented sample. The degree of orientation is greater than 95% as seen from the relative intensity of methyl signals arising from lipids oriented at 0° (6 kHz) and at 90° (3 kHz). In such sample, an accurate measurement of methylene and methyl 2H quadrupolar splittings could be realised (Table 1). 2H quadrupolar splittings were consistent with values found with liposomes (MLVs) (ΔνQ(0°) = 2ΔνQ(90°)). Furthermore, the phase transition temperature of the lipid mixture was not affected. Liposome-like dynamic behaviour of oriented lipids in this sample was confirmed by T2e investigations of DMPCd54 acyl chains (data not shown). Overall, bilayers mechanically oriented between glass plates and prepared from GVs deposition provide a good model of lipid membranes.
3.2 Preparation of proteolipidic GVs and oriented bilayers
It was shown recently that the electroformation technique could be adapted for the reconstitution of membrane proteins into giant unilamellar vesicles [17]. The protocol was adapted for the deuterium solid-state NMR study of phospholipids in interaction with K. pneumoniae OmpA (KpOmpA). Various protein-to-lipid molar ratios were investigated: 1:500, 1:150, 1:100 and 1:50. Trials to incorporate a higher proportion of proteins failed to provide well-oriented samples at the end of the protocol. Experiments were performed with the same lipid mixture DMPCd54/DLPC (25:75 mol/mol). DLPC (acyl chain with 12 carbons) was chosen to provide a membrane hydrophobic thickness close to KpOmpA membrane domain [23] and 25% of chain deuterated DMPC (acyl chain with 14 carbons) was included to allow 2H NMR experiments to be performed. The reinsertion of KpOmpA into this lipid mixture was confirmed by sucrose gradient density centrifugation (observation of a well defined proteolipid band, with a lipid to protein ratio equal to the desired one). The reconstitution of kpOmpA in its native state was confirmed by SDS-PAGE electrophoresis migration assay [23]. We investigated the effects of KpOmpA on the structure and dynamics of DMPCd54 in this lipid mixture. Fig. 3a shows the 2H powder pattern spectrum of DMPCd54/DLPC (25:75 mol/mol), with KpOmpA at a protein to lipid molar ratio of 1/50, recorded at 30 °C, which could be compared with the pure lipid spectrum (Fig. 1b). As the protein concentration was increased, 2H powder spectra were blurred without a measurable increase in the average orientational order parameters. This can be explained by the ability of lipid bilayer to match with hydrophobic thickness of KpOmpA systems without much change in the average orientational order parameters of the lipid acyl chains (no significant hydrophobic mismatch with the C12 acyl chains of DLPC). Preliminary study of T2e 2H relaxation on GV suspensions seems to indicate that the acyl chains dynamics of DMPCd54, is affected by KpOmpA (leading to a reduction in T2e by a factor 2, going from 1 ms to 450 μs in the presence of protein), thus explaining the loss of resolution.
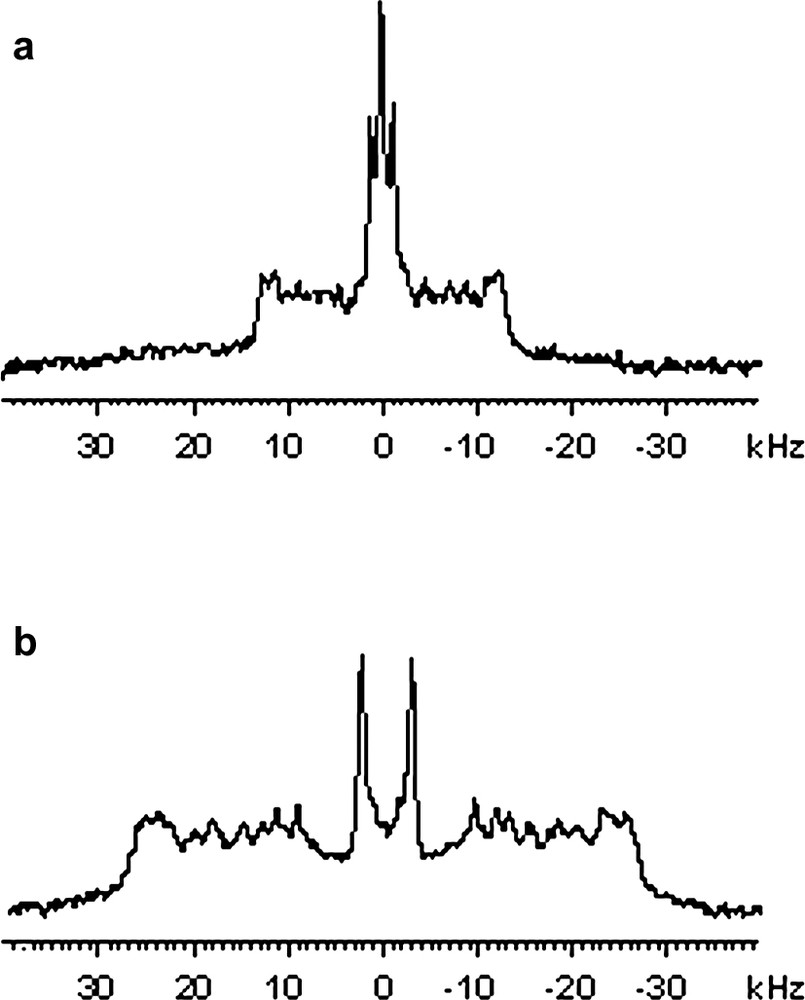
2H NMR spectra of bilayers composed of 2H-labelled DMPCd54/DLPC (25:75 mol/mol) with KpOmpA at a protein to lipids molar ratio of 1:50, 303 K. (a) GVs in aqueous solution, (b) Oriented bilayers, bilayer normal aligned with magnetic field.
In order to study the structure and global dynamic behaviour of KpOmpA in membrane bilayers, one may use 15N relaxation data of protein amide resonance using oriented lipid bilayers, as was performed previously with bacteriorhodopsin [14]. A prerequisite for these experiments is to prepare well-oriented samples at a high protein to lipid ratio. Oriented samples of DMPCd54/DLPC (25:75 mol/mol) containing KpOmpA have thus been prepared. Our first attempts to prepare oriented bilayers starting from SUVs containing KpOmpA, and fusion of these SUV by several cycles of dehydration–rehydration on the glass plates (our classical procedure), failed to provide oriented spectra of good quality, particularly at high protein to lipid ratio, and we tried a new procedure starting from giant proteo-liposomes. Giant vesicles suspension of KpOmpA/DMPCd54-DLPC (1:50 mol/mol), was gently submitted to a ‘bath sonication’ and directly deposited onto clean glass plates. This procedure resulted in fairly well oriented sample as shown in Fig. 3b. The degree of orientation was estimated to about 80% (from the relative intensity of 0° vs. 90° methyl signals of deuterated DMPC) and confirmed by analysis of the 31P spectra, for which it was observed that both DLPC and DMPC molecules were well oriented (data not shown). The gain in resolution on the oriented spectra (Fig. 3b) revealed that KpOmpA did not modify the quadrupolar splitting and hence the order parameter on hydrocarbon chains of DMPCd54 in DMPCd54/DLPC (25:75, mol/mol) oriented lipid bilayer, as it has already been observed for the proteo-GVs (Fig. 3a).
Giant proteolipidic vesicles prepared by the electroformation technique was thus shown to be a useful tool for 2H NMR characterisation of lipid membranes. Contrary to LUVs obtained by many standard protocols of proteoliposome formation such as detergent dialysis, detergent removal with bio-Beads or reverse phase evaporation, GVs can be studied in excess water and provide high quality powder spectra because of their large diameters. The spectra are as good as those obtained from MLVs, and even slightly more resolved, due to the partial orientation at 90° with respect to the magnetic field of bilayer normal. GVs were shown to be good intermediates in the preparation of oriented bilayers and actually were the only way for us to obtain well oriented lipid bilayers at high KpOmpA to lipid ratio (1/50 mol:mol, which converts into 74/100 weight ratio). High quality oriented bilayers is a prerequisite for the analyses of membrane proteins structure and dynamics by 15N NMR using 15N chemical shift anisotropies and 15N–1H dipolar couplings as structural constraints [2,3]. In this context, these results will allow us to study the dynamics of β-barrel proteins by analysing 15N relaxation properties of peptide planes and to compare them with those of α-helical proteins such as bacteriorhodopsin, which we have recently studied [14].
Acknowledgements
The CIPF (P. Fabre) is acknowledged for providing the pVALP40 plasmid of KpOmpA.
Vous devez vous connecter pour continuer.
S'authentifier