1 Introduction
Mesoporous materials have been used technically as adsorbents, catalysts and catalyst supports because of their high surface area. The first synthesis of an ordered mesoporous material was described in the literature in 1971 [1]. However due to a lack of analysis, the remarkable features of this material were not recognized [2]. The mesoporous material based on silica has been investigated for practical applications. In particular, the success of Mobil scientists in the discovery of a new family of mesoporous molecular sieves with hexagonal ordering of pore system (MCM-41) has been followed by an enormous amount of research worldwide [3]. MCM-41 with uniformly sized nanochannels, tunable pore size (1–10 nm) and high surface area (∼1000 m2/g) have been a focus of recent applications of catalysts, catalytic supports, molecular separation, chemical sensors, electronic and optical devices, and advanced materials [4].
12-Tungstophosphoric acid (TPA), as a Keggin type heteropoly acid, has attracted considerable attention as acid catalyst in organic transformations because of its high Brønsted acidity and thermal stability [5]. However, the use of bulk TPA has some disadvantages such as low surface area and high solubility in polar solvents which have reduced its application in some organic transformations. The use of TPA in supported form, especially over high surface area materials has attracted much attention. A TPA cluster with ∼1.2 nm diameter allows it to be introduced inside the MCM-41 pores [6]. Therefore, the large surface area of the supported TPA on MCM-41 makes it potential solid acid catalyst. Besides that, its large pore size plays a major role to overcome small pore obstruct of bulky organic molecules. MCM-41 supported 12-tungstophosphoric acid (TPA/MCM-41) has been used as catalyst in many organic transformations [5,7,8].
Quinoxaline and its derivatives exhibit various biological activities such as antiviral, antibacterial, anti-inflammatory and as kinase inhibitors [9]. A number of synthetic methodologies have been developed for the preparation of substituted quinoxalines. The most common method is the condensation of 1,2-dicarbonyles and o-phenylenediamines in the presence of an acidic catalyst such as SSA [10], PEG-400 [11] ZrO2/Ga2O3/MCM-41 [12], Ga(OTf)3 [13], cellulose sulfuric acid [14], ZrCl4 [15], NaHSO3 [16], NH4Cl [17] and ultrasonic irradiation [18]. However, many of these methods suffer from some limitations such as hard reaction conditions, low yield and long reaction times.
In continuation of our studies on the application of solid-supported reagents in organic transformations [7,19–21], in the present study, we report preparation, characterization and catalytic application of TPA/MCM-41 in the reaction of diketones and 1,2-phenylenediamines for the synthesis of quinoxalines (Scheme 1).

2 Experimental
2.1 Materials and methods
All chemicals were commercial products. All reactions were monitored by TLC and all yields refer to isolated products. 1H and 13C NMR spectra were recorded in CDCl3 on a Bruker (DRX-500 AVANCE) 500 MHz spectrometer. Infrared spectra of the catalysts and reaction products were recorded on a Bruker FT-IR Equinax-55 spectrophotometer in KBr with absorption in cm−1. X-ray diffraction (XRD) patterns were recorded on a Bruker D8 ADVANCE X-ray diffractometer using nickel filtered Cu Kα radiation. The morphology was studied using a Philips XL30 scanning electron microscopy.
2.2 Preparation of MCM-41
The synthesis of nano-sized MCM-41 was carried out using tetraethyl orthosilicate (TEOS) as Si source, cetyltrimethylammonium bromide (CTAB) as template and ammonia as pH control agent with the gel composition of SiO2:CTAB:NH4OH:H2O = 22.5:2.74:53.5:11.11.
In a typical procedure, CTAB (1 g, 2.74 mmol) was dissolved in deionised water (200 mL). After 10 min, TEOS (5 mL) was slowly added to the solution under continuous stirring for 0.5 h at 70 °C. Then the pH of the solution was adjusted to 10.5 by adding 12 wt.% ammonia solution for 2 h (∼4 mL). The mixture was allowed to cool to room temperature and was stirred for 12 h. The gel was recovered by centrifuge and washed with distilled water (2 × 2 mL). The obtained solid was dried in oven at 120 °C for 2 h and calcined in air at 550 °C for 4 h.
2.3 Preparation of 10 wt.% TPA/MCM-41
10 wt.% TPA/MCM-41 catalyst was prepared by mixing of TPA (10 mg), MCM-41 (90 mg) and deionised water (15 mL). The resulting suspension was stirred for 12 h followed by evaporation to dryness. The solid was dried at 120 °C for 2 h, powdered and calcined at 550 °C in air for 4 h.
2.4 General procedure for the synthesis of quinoxalines
A mixture of diketone (1 mmol) and diamine (1 mmol) in the presence of 10 wt.% TPA/MCM-41 (15 mg, 0.05 mol% TPA) was stirred at room temperature in EtOH for an appropriate time. The progress of the reaction was followed by TLC using 5% EtOAc in n-hexane as eluent. After completion of the reaction, the catalyst was recovered and washed with EtOH (2 × 2 mL). The solvent was evaporated and the product was obtained in high purity. Further purification was achieved by column chromatography on silica gel using EtOAc:n-hexane, 5:95 as eluent. The pure quinoxalines were obtained in 82–99% yields (Table 2).
Synthesis of quinoxalines by the reaction of 1,2-phenylenediamines and 1,2-diketones in the presence of 10 wt.% TPA/MCM-41 at room temperature in EtOH.
Entry | Ar | Diamine (II) | Time (min) | Yield (%) |
1 | 0.25 | 99 | ||
2 | 0.25 | 90 | ||
3 | 390 | 91 | ||
4 | 300 | 93 | ||
5 | 40 | 96 | ||
6a | 0.25 | 99 | ||
7a | 0.25 | 91 | ||
8a | 60 | 91 | ||
9a | 180 | 88 | ||
10 | 88 | 86 | ||
11 | 28 | 82 |
a The reaction was carried out in EtOAc as solvent.
2.5 Physical and spectroscopic data for selected compound
2.5.1 2,3-Diphenylquinoxaline (Table 2, entry 1)
White solid, mp 127–129 °C (Lit. [22] 126–127 °C); IR (Neat): υmax (cm−1): 3057, 1542, 1520, 1478, 761, 729; 1H NMR (400 MHz, CDCl3): δ (ppm) = 7.36–7.40 (m, 6H), 7.56 (dd, 4H, J = 8, 1.8 Hz), 7.80 (dd, 2H, J = 6.4, 3.6 Hz), 8.21 (dd, 2H, J = 6.4, 3.2 Hz); 13C NMR (100.62 MHz, CDCl3): δ (ppm) = 128.30, 128.83, 129.24, 129.86, 129.99, 139.10, 141.26, 153.50.
2.5.2 6-Methyl-2,3-diphenylquinoxaline (Table 2, entry 2)
White solid, mp 115–117 °C (Lit. [22] 116–117 °C); IR (Neat): νmax (cm−1): 3055, 2920, 1620, 1555, 1484, 1344, 880, 695, 622; 1H NMR (500 MHz, CDCl3): δ (ppm) = 2.6 (S, 3H), 7.36–7.56 (m, 10H), 7.63 (d, 1H, J = 8.4 Hz), 7.99 (S, 1H), 8.1 (d, 1H, J = 8.5 Hz); 13C NMR (125.77 MHz, CDCl3): δ (ppm) = 22.35, 28.48, 128.66 (4 C), 129.06, 129.120, 129.158, 130.27 (2 C), 130.29 (2 C), 132.73, 139.69, 140.161, 140.91, 141.75, 153.015, 153.76.
2.5.3 6-Benzoyl-2,3-Diphenylquinoxaline (Table 2, entry 3)
White solid, mp 151–153 °C (Lit. [18] 139–140 °C); IR (Neat): υmax (cm−1): 3057, 2922, 1659, 1600, 1598, 1444; 1H NMR (400 MHz, CDCl3): δ (ppm) = 7.26–7.33 (m, 6H), 7.44–7.50 (m, 7H), 7.82–7.84 (m, 2H), 7.83 (dd, 2H, J = 8, 1.4 Hz), 8.21–8.22 (m, 2H), 8.47 (s, 1H); 13C NMR (100.62 MHz, CDCl3): δ (ppm) = 128.41, 128.55, 129.21, 129.36, 129.70, 129.79, 129.92, 130.16, 132.46, 132.85, 137.18, 138.36, 138.52, 138.58, 140.19, 142.92, 154.63, 155.14, 195.82.
2.5.4 6-Nitro-2,3-diphenylquinoxaline (Table 2, entry 4)
White solid, mp 193–194 °C (Lit. [22] 192–193 °C); IR (Neat): υmax (cm−1): 3055, 2922, 1615, 1515, 1500, 1469, 1446, 1340; 1H NMR (500 MHz, CDCl3): δ (ppm) = 7.39–7.48 (m, 6H), 7.60 (t, 4H, J = 6.5 Hz),8.33 (d, 1 H, J = 9.1 Hz), 8.56 (dd, 1H, J = 9.1, 2.2 Hz), 9.19 (d, 1H, J = 2.3 Hz); 13C NMR (125.77 MHz, CDCl3): δ (ppm) = 123.73, 126.06, 128.89, 130.07, 130.20, 130.26, 130.33, 131.192, 138.47, 138.54, 140.42, 144.02, 148.32, 156.13, 156.75.
2.5.5 2,3-Dihydro-5,6-diphenylpyrazine (Table 2, entry 5)
White solid, mp 160–163 °C (Lit. [23] 163 °C); IR (Neat): υmax (cm−1): 3050, 2945, 1560, 1552, 1443; 1H NMR (500 MHz, CDCl3): δ (ppm) = 3.73 (s, 4H), 7.28 (t, 4H, J = 7.5 Hz), 7.34 (t, 2H, J = 7 Hz), 7.43 (d, 4H, J = 7.5 Hz); 13C NMR (125.77 MHz, CDCl3): δ (ppm) = 46.25, 128.33, 128.54, 130.05, 138.21, 160.73.
2.5.6 2,3-Difuran-2-yl-quinoxaline (Table 2, entry 6)
Pale brown solid, mp 132–134 °C (Lit. [24] 129–130 °C); IR (Neat): υmax (cm−1): 3107, 1571, 1535, 1479, 1163, 1058; 1H NMR (500 MHz, CDCl3): δ (ppm) = 6.59–6.60 (m, 2 H), 6.7 (d, 2 H, J= 3.31 Hz), 7.6 (d, 2H, J = 1.02 Hz), 7.77–7.79 (m, 2 H), 8.15–8.18 (m, 2 H); 13C NMR (125.77 MHz, CDCl3): 112.35, 113.43, 129.56, 130.83, 141.074, 143.09, 144.66, 151.25.
2.5.7 6-Methyl-2,3-difuran-2-yl-quinoxaline (Table 2, entry 7)
Pale brown solid, mp 118–120 °C (Lit. [18] 112–114 °C); IR (Neat): υmax (cm−1): 3110, 2900, 1618, 1569, 1490, 1350, 1147, 1062; 1H NMR (400 MHz, CDCl3): δ (ppm) = 2.52 (s, 3H), 6.48–6.49 (m, 2H), 6.54–6.56 (m, 2H), 7.51 (dd, 2H, J = 8.6, 1.8 Hz), 7.54–7.55 (m, 1H), 7.84 (s, 1H), 7.95 (d, 1H, J = 8.4 Hz); 13C NMR (100.62 MHz, CDCl3): δ (ppm) = 21.95, 111.87, 111.89, 112.58, 112.83, 127.99, 128.64, 132.80, 139.12, 140.74, 141.14, 141.87, 142.62, 144.01, 144.14, 150.92.
2.5.8 6-Benzoyl-2,3-difuran-2-yl-quinoxaline (Table 2, entry 8)
Pale brown solid, mp 118–120 °C (Lit. [13] 132–134 °C); IR (Neat): υmax (cm−1): 3135, 1654, 1597, 1154, 1065; 1H NMR (400 MHz, CDCl3): δ (ppm) = 6.60–6.63 (m, 2H), 6.76–6.79 (m, 2H), 7.53–7.57 (m, 3H), 7.64–7.69 (m, 2H), 7.90–7.92 (m, 2H), 8.27 (d, 2H, J = 1.6 Hz), 8.50 (t, 1H, J = 1.2 Hz); 13C NMR (100.62 MHz, CDCl3): δ (ppm) = 112.09, 112.22, 113,62, 114,26, 128.55, 129.59, 130.11, 130.29, 132.29, 132.84, 137.11, 138.55, 139.54, 142.42, 143.47, 143.97, 144.56, 144,89, 150.52, 195.67.
2.5.9 6-Nitro-2,3-difuran-2-yl-quinoxaline (Table 2, entry 9)
Orange solid, mp 171–173 °C (Lit. [18] 164–166 °C); IR (Neat): υmax (cm−1): 3129, 1617, 1563, 1520, 1467, 1300, 1160, 1058; 1H NMR (400 MHz, CDCl3): δ (ppm) = 6.63–6.66 (m, 2H), 6.85 (dd, 1H, J = 3.4, 0.6 Hz), 6.91 (dd, 1H, J = 3.4, 0.6 H), 7.69–7.71 (m, 2H), 8.25 (d, 2H, J = 9.2 Hz), 8.51 (dd, 2H, J = 9.2, 3.2 Hz), 9.04 (d, 1H, J = 3.2); 13C NMR (100.62 MHz, CDCl3): δ (ppm) = 112.31, 112.44, 114.51, 115.37, 123.66, 125.37, 130.47, 139.26, 143.02, 144.23, 144.73, 145.01, 145.49, 147.95, 149.40, 150.17.
2.5.10 2,3-Bis(4-methoxyphenyl)quinoxaline (Table 2, entry 10)
White solid, mp 151–152 °C (Lit. [25] 151–152 °C); IR (Neat): υmax (cm−1): 3010, 3090, 1605, 1511, 1394, 1346, 1240, 1026; 1H NMR (500 MHz, CDCl3): δ = 3.8 (S, 6 H), 6.91 (d, 4 H, J = 6.73 Hz), 7.53 (d, 4H, J = 6.63 Hz),7.76(dd, 2 H), 8.16 (dd, 2 H); 13C NMR (125.77 MHz, CDCl3): δ = 55.74, 114.21, 129.44, 129.95, 131.68, 132.17, 141.51, 153.46, 160.60.
2.5.11 6-Methyl-2,3-bis(4-methoxyphenyl)quinoxaline (Table 2, entry 11)
White solid, mp 123–126 °C (Lit. [26] 124–126 °C); IR (Neat): υmax (cm−1): 3050, 2900, 1655, 1604, 1511, 1343, 1246, 1027; 1H NMR (400 MHz, CDCl3): δ (ppm) = 2.52 (s, 3H), 3.75 (s, 6H), 6.79 (d, 4H, J = 7.2 Hz), 7.40 (d, 4H, J = 7.2 Hz), 7.47 (d, 1H, 7.6 Hz), 7.84 (s, 1H), 7.93 (d, 1H, 7.6 Hz); 13C NMR (100.62 MHz, CDCl3): δ (ppm) = 21.89, 55.31, 113.74, 127.88, 128.53, 131.20, 131.24, 132.87, 139.54, 140.00, 141.14, 152.17, 152.90, 160.01.
3 Result and discussion
3.1 Catalyst characterization
The structure of TPA/MCM-41 is well known and has been reported in many papers. However, the small difference in the procedure of preparation of the MCM-41 still requires some characterization experiments.
The morphology of the MCM-41 was studied by scanning electron microscopy (SEM) (Fig. 1). SEM image shows the MCM-41 as agglomerated nanoparticles with the size range less than 100 nm.
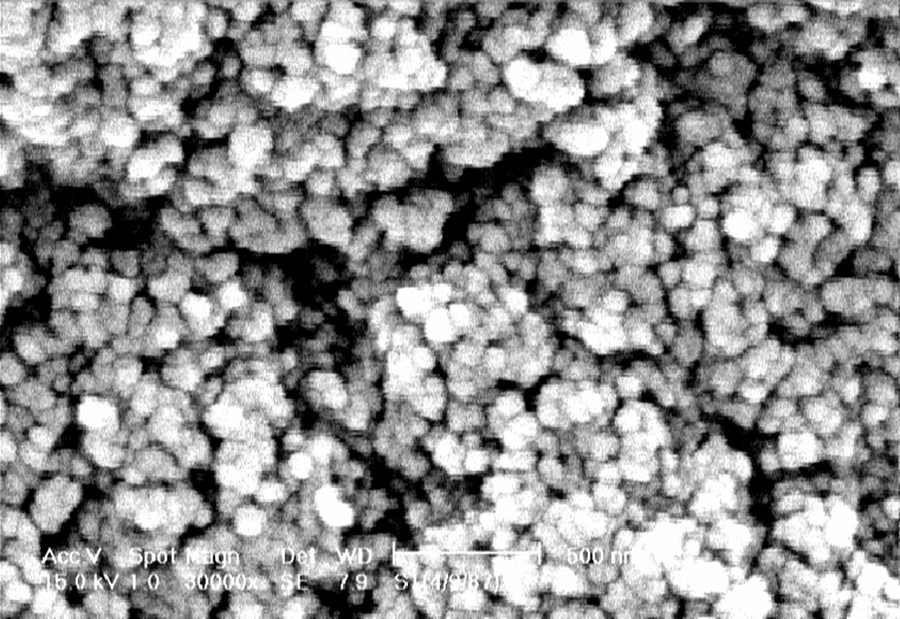
SEM image of MCM-41.
The FT-IR spectra of TPA, MCM-41 and TPA/MCM-41 with 10 wt.% loading amounts of TPA are shown in Fig. 2. The bulk TPA shows four peaks at 1080, 981, 887 and 806 cm−1 which are assigned to the stretching vibrations of P-Oa, W-Od, W-Ob-W and W-Oc-W, respectively. As shown in the spectra of TPA/MCM-41, two characteristic peaks of TPA (887, 1080 cm−1) are overlapped over the main peaks of MCM-41 (812 and 1084 cm−1). However, the peak at 806 cm−1 was observed in the TPA/MCM-41 spectrum without any change compared to bulk TPA, and the peak at 960 cm−1 was observed in this spectrum corresponding to the peak at 981 cm−1 for the bulk TPA spectrum. These results confirm presence of TPA with Keggin structure on the MCM-41.
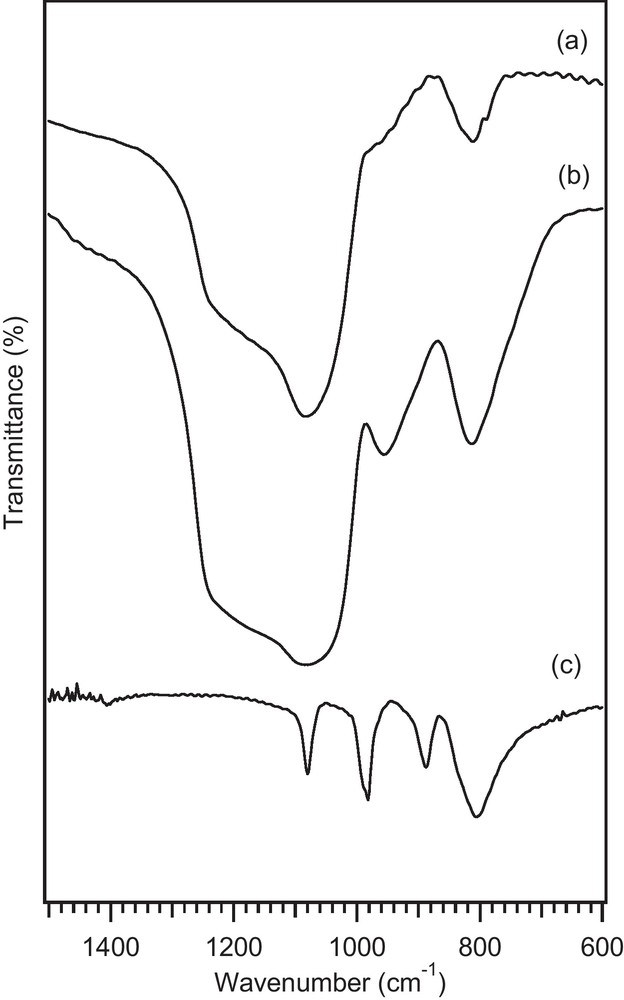
FT-IR spectra of (a) MCM-41; (b) 10 wt.% TPA/MCM-41, (c) pure TPA.
The presence and dispersion state of TPA on the MCM-41 were investigated by XRD technique (Fig. 3). XRD patterns of the catalysts with different loading amount of TPA were compared with MCM-41 and bulk TPA. The characteristic peaks of the MCM-41 are at 2θ = 2.3, 4 and 4.6°. As shown in XRD patterns of TPA/MCM-41 (Fig. 3b–d), supporting of MCM-41 with TPA can affect the width and intensity of these characteristic peaks. These results suggest that the long-range order of MCM-41 was decreased when increasing the loading amount of TPA.
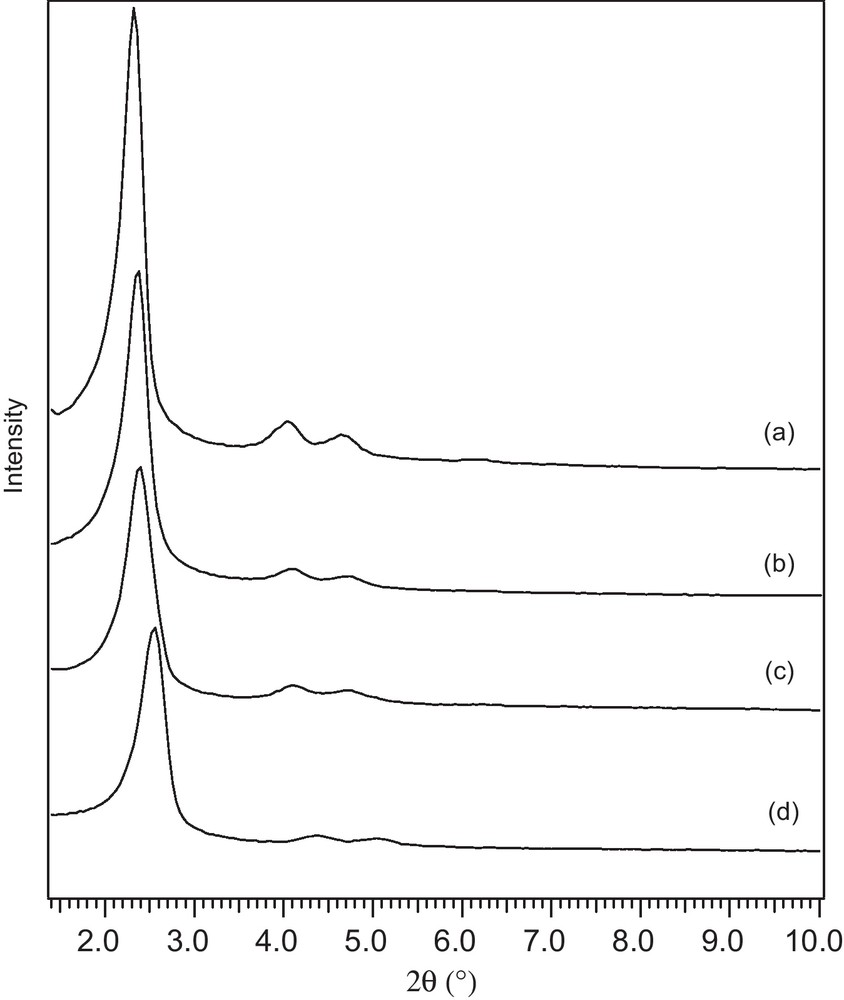
The low angle XRD patterns of (a) MCM-41; (b) 5 wt.% TPA/MCM-41, (c) 10 wt.% TPA/MCM-41, (d) 15 wt.% TPA/MCM-41.
The dispersion state of TPA was investigated by high angle XRD (Fig. 4). As shown in Fig. 4a, no diffraction peaks of TPA appear in the spectra of catalyst of 10 wt.% TPA/MCM-41. This result indicates that the TPA is highly dispersed on the 10 wt.% TPA/MCM-41.
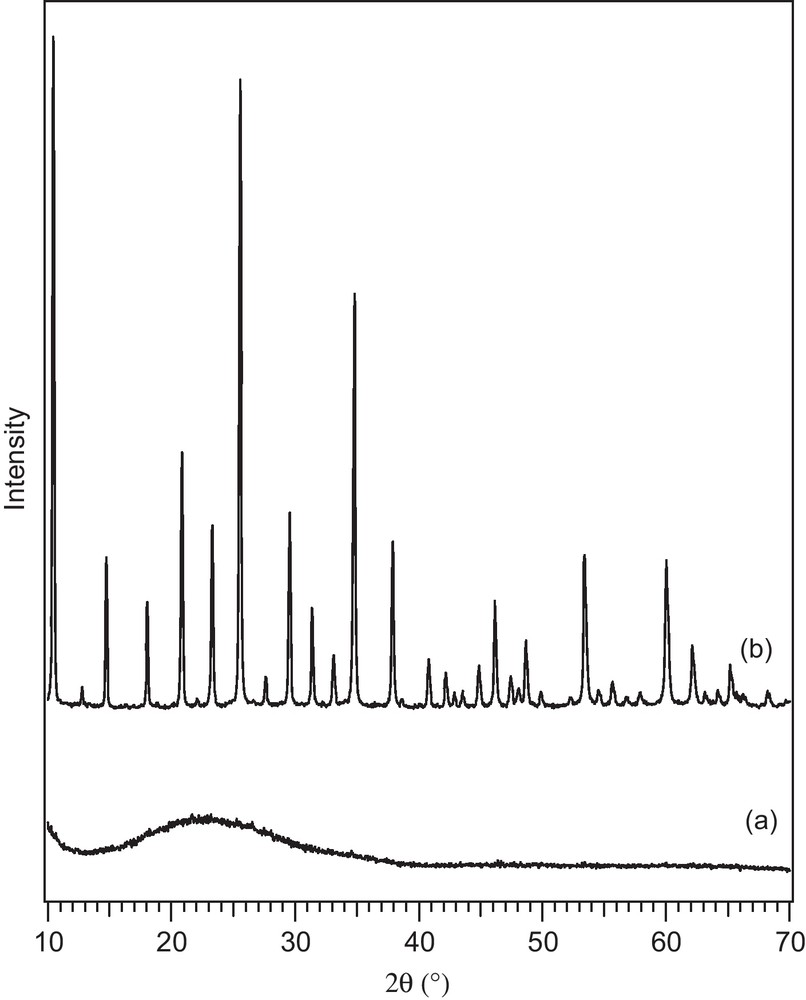
The high angle XRD patterns of (a) 10 wt.% TPA/MCM-41, (b) TPA.
3.2 Catalytic activity of TPA/MCM-41 in the synthesis of quinoxalines
In order to investigate the catalytic activity of TPA supported on the MCM-41 nanoparticles in the synthesis of quinoxalines, the reaction of various types of 1,2-diketones and 1,2-phenylenediamines were studied in the presence of TPA/MCM-41.
Initially, the optimization experiments were performed in the reaction of 1,2-phenylenediamine (1 mmol) and benzil (1 mmol) as the model reaction and the results are shown in Table 1.
Optimization of reaction conditions for the synthesis of quinoxalines in the presence of catalytic amount of TPA/MCM-41.
Entry | Catalyst | Solvent | Catalyst amount (mg/mol%) | Time (min) | Yield (%) |
1 | 5% TPA/MCM-41 | EtOH | 15/0.026 | 2 | 98 |
2 | 10% TPA/MCM-41 | EtOH | 15/0.052 | 0.25 | 99 |
3 | 15% TPA/MCM-41 | EtOH | 15/0.078 | 2 | 94 |
4 | 10% TPA/MCM-41 | EtOH | 10/0.035 | 5 | 90 |
5 | 10% TPA/MCM-41 | EtOH | 20/0.07 | 10 | 95 |
6 | 10% TPA/MCM-41 | EtOAc | 15/0.052 | 1 | 99 |
7 | 10% TPA/MCM-41 | CH3CN | 15/0.052 | 20 | 99 |
8 | 10% TPA/MCM-41 | CH2Cl2 | 15/0.052 | 44 | 92 |
9 | 10% TPA/MCM-41 | CHCl3 | 15/0.052 | 21 | 99 |
To investigate the effect of the loading amount of TPA on the catalytic activity of TPA/MCM-41, the model reaction was performed in the presence of 15 mg of the catalyst with 5–15 wt.% TPA (Table 1, entries 1–3). The results show that 10 wt.% TPA/MCM-41 has the best catalytic activity.
In order to optimize the amount of the 10 wt.% TPA/MCM-41, the model reaction was performed with various amounts of the catalyst and in terms of reaction time and yield of the product, 15 mg of the catalyst (0.052 mol%) for the reaction of 1 mmol benzil in the model reaction was selected as the best amount (Table 1, entries 3–5).
To investigate the effect of solvent in the reaction, the model reaction in the presence of 15 mg 10 wt.% TPA/MCM-41 was carried out in the various solvents (Table 1, entries 3, 7–11). The best solvent is EtOH with the best yield and time for the reaction (Table 1, entry 3).
Efficiency of the catalyst in the reaction was also examined in a blank reaction, without catalyst, in EtOH at room temperature and reflux condition and the reactions were completed after 20 h and 3.5 h, respectively.
The scope and generality of this catalytic reaction is illustrated with respect to the reaction of different 1,2-phenylenediamines with 1,2-diketones and the results are summarized in Table 2.
As shown in Table 2, the reaction of 1,2-phenylenediamine with benzil was carried out in the presence of 15 mg 10 wt.% TPA/MCM-41 (0.052 mol%) at room temperature in EtOH and the corresponding quinoxaline was obtained in 99% yield. The reaction of various types of 1,2-phenylenediamines and 1,2-diketones with both electron donating and electron withdrawing substituents were carried out and the corresponding quinoxalines were obtained in high to excellent yields (Table 2, entries 2–11).
The workup and catalyst recovery is very easy. After the completion of the reaction (monitored by TLC, eluent; EtOAc:n-hexane, 5:95), EtOH was added to the reaction mixture and the catalyst was separated by centrifuge and washed with EtOH. After evaporation of EtOH the crude product was obtained in high purity. Further purification was achieved by column chromatography on silica gel using EtOAc:n-hexane, 5:95 as eluent.
To study the reusability of the TPA/MCM-41, the recovered catalyst from the model reaction was washed with EtOH and dried in an oven at 120 °C for 2 h. The recovered catalyst was reused in the same reaction three times. The reaction proceeded smoothly with a yield of 99–98% (Table 3). Although a serious problem of heteropoly acid catalysts is coke deposition during the reaction process, the obtained results indicate that the catalyst does not appreciable change in its activity and shows only a slight decrease in the yield after first run.
Reusability of 10 wt.% TPA/MCM-41 in the reaction of 1,2-phenylenediamine and benzil.
Run | Time (s) | Yield (%) |
1 | 15 | 99 |
2 | 15 | 98 |
3 | 15 | 98 |
4 | 15 | 98 |
The efficiency of 10 wt.% TPA/MCM-41 was also compared with other similar heterogeneous catalytic systems in the model reaction (Table 4). It is noteworthy that many heterogeneous catalysts listed in Table 4 are not available and their preparation requires harsh condition. Nonetheless, advantages of solid acid catalysts especially their reusability makes them attractive for synthetic methodology.
Comparison of the catalytic activity of 10 wt.% TPA/MCM-41 with other heterogeneous catalysts in the synthesis of quinoxalines.
Entry | Catalyst | Catalyst amount | Solvent, Reaction condition | Reaction time (min) | Yield (%) | Ref. |
1 | 10% TPA/MCM-41 | 0.052 mol% | EtOH, r.t. | 0.25 | 99 | – |
2 | Montmorillonite K-10 | 10 mol% | H2O, r.t. | 150 | 100 | [27] |
3 | Polyaniline sulfate salt | 5 wt.%a | H2O, r.t. | 40 | 95 | [28] |
4 | SbCl3/SiO2 | 2.5 mol% | CH3OH, r.t. | 5 | 100 | [26] |
5 | Zn2+-K10-Clay | 125 mg | H2O/CH3CN, r.t. | 150 | 89 | [29] |
6 | 17%ZrO2/4%Ga2O3/MCM-41 | 200 mg | CH3CN, r.t. | 120 | 97 | [12] |
7 | Cellulose sulfuric acid | 10 mg | EtOH, r.t. | 60 | 93 | [14] |
8 | Sulfated titania | 100 mg | EtOH, r.t. | 5 | 99 | [30] |
9 | Sulfated titania | 100 mg | EtOH, MW | 1 | 99 | [31] |
10 | Acidic alumina | 200 mg | –, 80 °C | 2 | 96 | [32] |
a wt.% relative to dicarbonyl compound
However, the results of Table 4 show that the present catalyst in terms of catalyst amount, yield or reaction time is comparable with other heterogeneous catalyst.
4 Conclusion
In summary, we have demonstrated that TPA on the MCM-41 nanoparticles can be used as efficient and reusable catalyst for the synthesis of quinoxalines by the reaction of 1,2-phenylenediamines and 1,2-diketones. The simple experimental procedure, mild reaction conditions, ease of catalyst recovery and reusability are some other advantages of this method.
Acknowledgements
We are thankful to the Yazd University Research Council for partial support of this work.
Vous devez vous connecter pour continuer.
S'authentifier