1 Introduction
The mercury(II) halides, HgX2 with X = I, Br, Cl and F, form an amazing number of solid phases and adopt remarkably different crystal structures. In this contribution, we review the structures of these compounds known to date and their geometric relations. We also present the corresponding pressure–temperature phase diagrams. The structures of solid HgX2 fall into two very different classes: (i) semiconductor-type framework structures where the anions X form nearly ideal closest sphere packings, and (ii) structures built from linear or bent HgX2 molecules where the anions X form very distorted close packings.
HgI2 exhibits the largest diversity of structures. This compound has been extensively studied by many techniques in view of its opto-electronic properties and the technological interest has resulted in several hundreds of publications that have been reviewed in [1]. At ambient conditions, HgI2 adopts structures of both classes. The stable red phase forms a tetrahedral close-packing structure. It is used as room-temperature detector material for X- or γ-rays [2–4]. Besides, there exist two metastable forms at ambient conditions, an orange one of class (i) and a yellow one of class (ii). The known high-temperature and high-pressure phases are molecular. HgBr2 is also a candidate material for the detection of photons [5], but has been much less investigated in comparison to HgI2. At ambient pressure, this compound adopts a molecular class-(ii) structure, whereas at high pressure it forms a modulated CdI2-type class-(i) structure with octahedral and pyramidal coordinations of Hg. Several high-pressure phases with unknown structures also exist. For HgCl2, only molecular class-(ii) structures are known. Pure water-free HgF2 is difficult to prepare, and therefore its phase diagram is not known. At ambient conditions, it forms a fluorite-type (CaF2) structure built from corner-linked HgF8 cubes.
2 Mercuric iodide
2.1 Polymorphism of HgI2 at ambient conditions
Mercuric iodide (HgI2) is known to crystallize from various organic solvents and also by sublimation into three concomitant polymorphs whose colours are red, orange, and yellow [6]. At ambient conditions, the red phase is thermodynamically stable, while the orange and yellow phases are metastable and transform into the red phase when touched. The aspects and speeds of these transformations are different for the two forms. In the orange crystals, red nuclei develop into domains of irregular shape, which spread and transform a crystal of diameter 0.2 to 0.5 mm within hours or days while preserving the external shape. In contrast, in the yellow form, a sharp red front propagates from the contact point and consumes all of a crystal of dimensions 0.2 to 0.5 mm within a few seconds while the crystal may warp. From a 2-chloroehtanol solution, the yellow crystals are the first, and the red crystals the last to crystallize, as observed by optical microscopy [7]. Faster evaporation favours the formation of yellow crystals. Above 323 K, only yellow crystals are formed, whereas slow crystallization in a closed vessel gives only red, orange or red-orange composite crystals.
2.2 Crystal structures of the polymorphs at ambient conditions
The structures of the polymorphs of HgI2 at ambient conditions are shown in Fig. 1; Table 1 lists unit cell parameters and space group symmetries. All the structures of the red and the orange phases have in common a nearly ideal cubic closest packed (ccp) substructure of iodine atoms with 1/4 of the tetrahedral interstices occupied by Hg. The HgI4 tetrahedra are corner-linked and all Hg–I–Hg and I–Hg–I angles are close to tetrahedral. There is only one kind of Hg atom, i.e. all tetrahedra (but not all corners, i.e. iodine atoms) are symmetry-equivalent. It can be shown that six different structures with these properties, all of them tetragonal, can be derived from a ccp-array of anions (at least two such structures exist for the hexagonal hcp array with space group symmetries Pmc21 and Pbam; to our knowledge, they have never been observed experimentally). Four of these six structures are realized by HgI2; one of remaining structures has not (yet) been identified for HgI2 but is realized by ZnCl2; a sixth structure has never been observed. The stable red form consists of layers of corner-linked HgI4 tetrahedra [8,9], stacked by I···I contacts. The metastable orange crystals comprise three different crystal structures, all of which are built from corner-linked Hg4I10 supertetrahedra [10,11]. Two of them are end members with the maximum degree of order (MDO) of a polytypic layer structure; the third shows a three-dimensional diamantoid-type linkage (D). The two MDO structures are fractal complications of the stable red form of HgI2 obtained by replacing single tetrahedra by supertetrahedra. The geometries and anisotropic displacement parameters of the layers in MDO1 and MDO2 and the interlayer contacts are nearly identical in the two stackings [10]. The two MDO structures usually coexist and form a disordered crystal. A quantitative analysis of the stacking disorder by fitting a Markov chain model to the intensities of the corresponding rods of diffuse X-ray scattering suggests the presence of nearly equal volumes of the MDO structures with an average domain thickness of about five layers or 30 Å [10]. The third orange structure D (diamantoid) is also composed of corner-linked Hg4I10 supertetrahedra. However, the supertetrahedra are not linked into layers but into two interpenetrating four-connected three-dimensional networks [11]. This is the fractal complication of the structure of ZnCl2. Composite crystals containing all three supertetrahedral structures MDO1, MDO2 and D, twinned in three perpendicular directions resulting in a pseudo-cubic edifice, have also been observed [11].
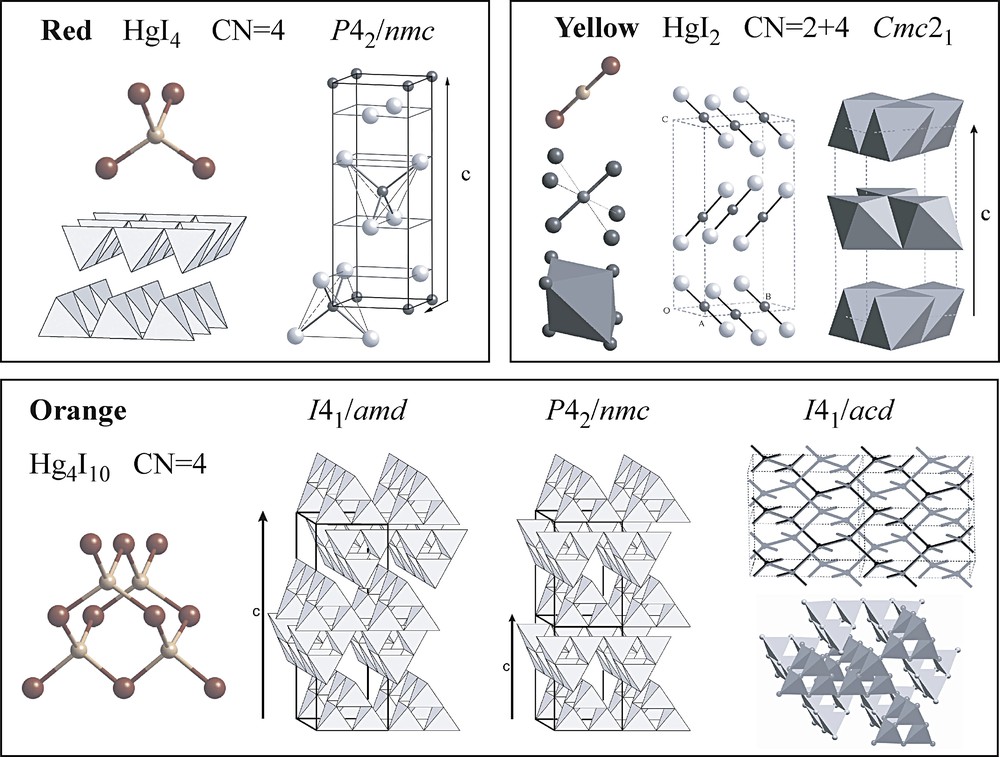
Structures of the polymorphs of HgI2 at ambient conditions. The red phase (III of Fig. 2) is thermodynamically stable. The orange phase comprises three different structures built of corner-shared Hg4I10 supertetrahedra. The two layered structures are end-members of a polytypic family with layer symmetry Pm2. In the third structure, the supertetrahedra form two interpenetrating three-dimensional networks.
Space-group symmetries and unit-cell dimensions of the solid phases of the halides HgX2. The subscript M indicates a metastable phase obtained at ambient conditions, ac stands for ambient conditions, HT for high temperature, HP for high pressure
Phase | Space group | a (Å) | b (Å) | c (Å) | β (°) | Reference | |
HgI2 | III at ac, red | P42/nmc | 4.361(5) | – | 12.450(7) | – | [9] |
HgI2 | orangeM MDO1 | I41/amd | 8.7860(9) | – | 24.705(9) | – | [10] |
HgI2 | orangeM MDO2 | P42/nmc | 8.7860(9) | – | 12.353(5) | – | [10] |
HgI2 | orangeM D | I41/acd | 12.3930(18) | – | 24.889(5) | – | [11] |
HgI2 | yellowM | Cmc21 | 4.734(1) | 7.408(2) | 13.943(3) | – | [7] |
HgI2 | II, yellowHT, 141(1) K | P21 | 8.0206(7) | 13.9239(7) | 4.4081(3) | 95.062(6) | [7] |
HgI2 | IV, red, 0.8(1) GPa | P42/nmc | 4.334(3) | – | 12.178(2) | – | [18] |
HgI2 | VI, yellHP, 1.8(1) GPa | P21/a | 13.948(3) | 5.343(2) | 5.113(2) | 106.51(2) | [18] |
HgI2 | VII, 11.0(1) GPa | hexag/trig | 8.441(2) | – | 12.073(3) | – | [18] |
HgBr2 | I at ac | Cmc21 | 4.628(2) | 6.802(2) | 12.476(2) | – | [24] |
HgBr2 | IV, 5,4(1) GPa | P3 | 6.7569(3) | – | 5.6589(9) | – | [27] |
HgBr2 | V, 10.7(1) GPa | hexag/trig | 6.787(1) | – | 11.130(2) | – | [27] |
HgCl2 | I, ac | Pnma | 12.776(4) | 5.986(3) | 4.333(2) | – | [28] |
HgCl2 | V, 572(1) K | cubic | 4.41(2) | – | – | – | [29] |
HgCl2 | IV, 1.7(1) GPa | P21/m | 12.378(2) | 5.711(1) | 8.369(1) | 91.01(5) | [29] |
HgCl2 | II, 2.2(1)GPa | Rm | 5.569(1) | – | 7.817(1) | – | [29] |
HgF2 | I, ac | Fmm | 5.54(1) | – | – | – | [32] |
In contrast to the red and orange forms, the structure of the yellow metastable (yellowM) polymorph at room temperature is molecular with linear I–Hg–I molecules (angle 180.4° not constrained by symmetry) [7,9]. Not surprisingly, the packing of the iodine atoms is very distorted. Closest-packed layers with six-coordinated spheres are stacked roughly midway between ccp and hcp. Therefore, the iodine substructure may be described as related to the cubic volume-centred bcc structure [7]. The coordination of mercury by iodine is very distorted octahedral 2+4, with two close and four considerably more distant neighbours.
2.3 Mechanisms of crystallization and transformation of HgI2
When crystallizing HgI2 at ambient conditions, rapid evaporation of the solvent is essential for the formation of the yellowM polymorph, indicating that the crystallization of this form is governed by kinetics. Raman spectra of an HgI2 solution in 2-chloroethanol indicate HgI2 to exist in the form of molecules [7]. Therefore, the observed crystallization sequence can be understood as a kinetic effect: the pre-existing molecules rapidly pack into the yellowM form, while the formation of HgI4 tetrahedra demands the breaking of bonds. On the other hand, it remains unclear why the orange structures are kinetically favoured and formed before the stable red structure. In both structures, there are four shortest Hg–Hg distances, but the Hg distribution in the orange forms is globular and somewhat more homogeneous than the planar distribution in the red form. It seems reasonable that the formation of clusters with limited dimensions is kinetically favoured over the formation of extended sheets. The choice between the different non-layer structures does not seem to be dominated by electrostatic interactions; we would expect the ZnCl2 structure, which is not realized by HgI2, to have the largest Madelung energy of the six tetrahedral structures mentioned above and thus to be the most ionic one. We also note that ZnI2 at ambient conditions forms the supertetrahedral D-structure. The transformations from orange to red presumably involve only movements of Hg atoms in an invariant ccp matrix of I. The thermal displacement parameters of Hg in the tetrahedral structures are indeed larger than those of iodine [10,11]. In contrast, the transformation from yellowM to red implies a complete rearrangement of the atoms.
2.4 Phase diagram of HgI2
The phase diagram of HgI2 has been investigated by many authors with different techniques. The somewhat controversial results are reviewed in [12]. A phase diagram compiled from all the various experimental observations is presented in Fig. 2. Five different phases (I, II, III, IV, VI) are reported in the moderate pressure–temperature range of p < 4 GPa, T < 700 K, the boundaries of which have been established by dilatometry (compression-curve method) [13], differential thermal analysis [14], Raman spectroscopy [15,16] and powder X-ray diffraction [17,18].
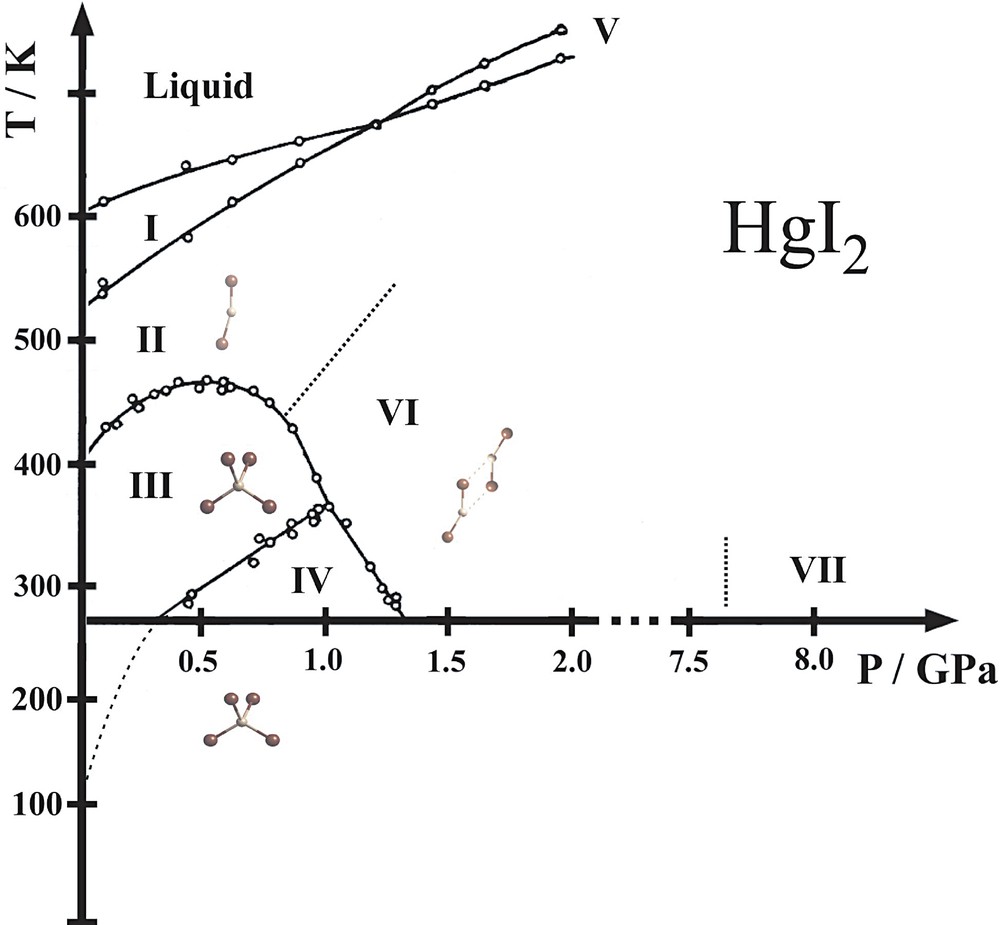
Phase diagram of HgI2. The phase boundaries have been established by differential thermal analysis (circles) [14]. The dotted line delimiting phases II and VI has been found by Raman spectroscopy [16]. The VI–VII transformation has been identified by X-ray diffraction, its boundary has been mapped only at room temperature [17]. The III–IV boundary is extrapolated from DTA data [14] to the transition point observed by X-ray diffraction at ambient pressure [18]. The corresponding structural motifs, if known, are shown in the stability regions of the phases. Powder X-ray diffraction data of phase VII indicate a hexagonal cell. No diffraction data exist for phases I and V and the existence of phase I has not been clearly established [12].
At 400 K and ambient pressure, the red form discussed above (phase III) undergoes a reconstructive transition to the yellow high-temperature phase II (yellowHT), which has been assumed to be identical to the yellowM polymorph obtained under ambient conditions [12]. However, we have observed the symmetry of the yellowHT phase to be P21, a subgroup of the space group Cmc21 of the yellowM structure. The two structures are closely related; both show similarly packed I–Hg–I molecules. The main difference consists in the molecules in yellowHT being bent and not linear (Fig. 3), with angles of 163(1)° and 157(1)° for the two molecules in the asymmetric unit. Electron diffraction and vibrational spectroscopy cannot rule out the existence of slightly bent molecules in the gas phase (165–180°); ab initio studies usually assume straight molecules [19]. The mechanisms of the III → II and the reverse II → III transitions are different. Upon heating, the transition occurs through surface nucleation while, upon cooling, it shows bulk nucleation [20]. The kinetics of the II–III transition is highly asymmetric. The growth rate of a newly formed crystal has been measured by the increase of a normalized integrated Bragg intensity with time, (ΔIBragg/IBragg) (s–1). For III→II, the growth rates of phase II at 402.9 and 404.0 K are 3.6 × 10–4 s–1 and 4.2 × 10–4 s–1, respectively. In contrast, for II→III, the growth rate at 381.0 K is much smaller, 1.8 × 10–5 s–1 [7]. The hysteresis of the transition is also asymmetric in the sense that phase II can be undercooled to room temperature, while the red phase can be overheated by only about 10 K above the transition temperature [7]. The II–III phase boundary presents the particularity of a maximum at about 0.5 GPa (Fig. 2). The volumetric data of Bridgman [13] show that there is no change of volume when crossing the transition line at the maximum of 0.5 GPa. At lower pressures, phase II is less dense than phase III, while at higher pressures phase II is denser. In addition, phase II presents a higher compressibility than phase III over the whole pressure range up to 1 GPa. This peculiar combination of higher compressibility of the denser phase is also observed for ice and water.
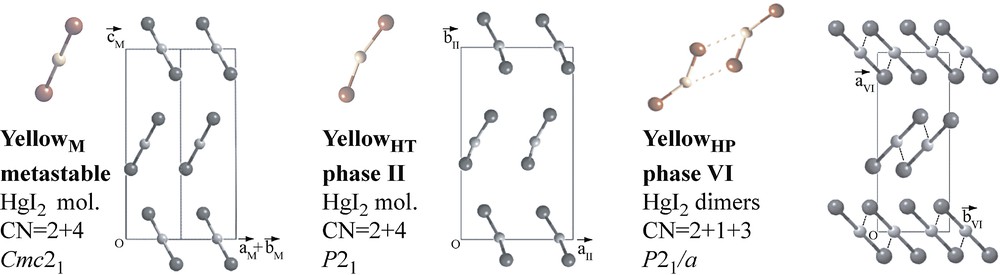
Structures of the yellow molecular phases of HgI2.
Several of the high-pressure structures have been determined, at least qualitatively [17,18,21]. Differential thermal analysis data [14] show the existence of a transition between the red phase III and another red phase IV at moderate temperatures and pressures (Fig. 2). Powder diffraction data collected as a function of pressure at room temperature show that the III–IV transformation is neither associated with a change of space group symmetry nor with a discontinuous change of volume of the unit cell. Instead, it shows two different ‘tetragonalities’ [18]: the slope of the change of the c/a ratio with pressure, and also with temperature, is discontinuous at the phase boundary, while c/a – changes sign. All observations obtained with various experimental techniques [18] may be explained by assuming that the III–IV transformation is an anti-isostructural transition [22].
Upon further increasing the pressure at room temperature, the red phase (III, IV) undergoes a transition to a yellowHP phase VI at about 1.2 GPa. At room temperature, phase VI is stable up to 7.7 GPa, at which pressure a transformation to phase VII occurs. Based on the first published diffraction data at high pressure [17], obtained with a sealed Mo tube and X-ray film, phase VI was proposed to be identical with phase II, and phase VII a polytypic variant of the CdI2 structure. Later Raman spectroscopy data disproved the identity of phases II and VI and the corresponding phase boundary was established [16]. The most recent diffraction experiments carried out with synchrotron radiation and area detector showed that the structure of phase VI is another variant of the yellow molecular phase [18,21], showing bent HgI2 molecules that form dimers (Fig. 3). Powder diagrams of phase VII at 11.0 GPa could be indexed with a hexagonal cell, c/a = 1.43 [18]. The data did not allow to solve the structure; for a CdI2-type structure c/a ~ 1.6.
3 Mercuric bromide
3.1 Phase diagram of HgBr2
At ambient conditions, the stable phase I of HgBr2 adopts the same structure as the yellow metastable polymorph of HgI2 [23,24]. At ambient pressure, no phase transitions have been reported, phase I being stable from 90 K, the lowest temperature investigated, to the melting temperature of 509 K. The application of pressure up to 4.5 GPa induces several transitions as revealed by dilatometry (compression-curve method) [25] and Raman spectroscopy [26]. Three phases (II, III, IV) have been reported in this pressure range (Fig. 4). The crystal structure of phase IV has recently been solved making use of synchrotron data, and an additional phase V above 9 GPa was identified [27].
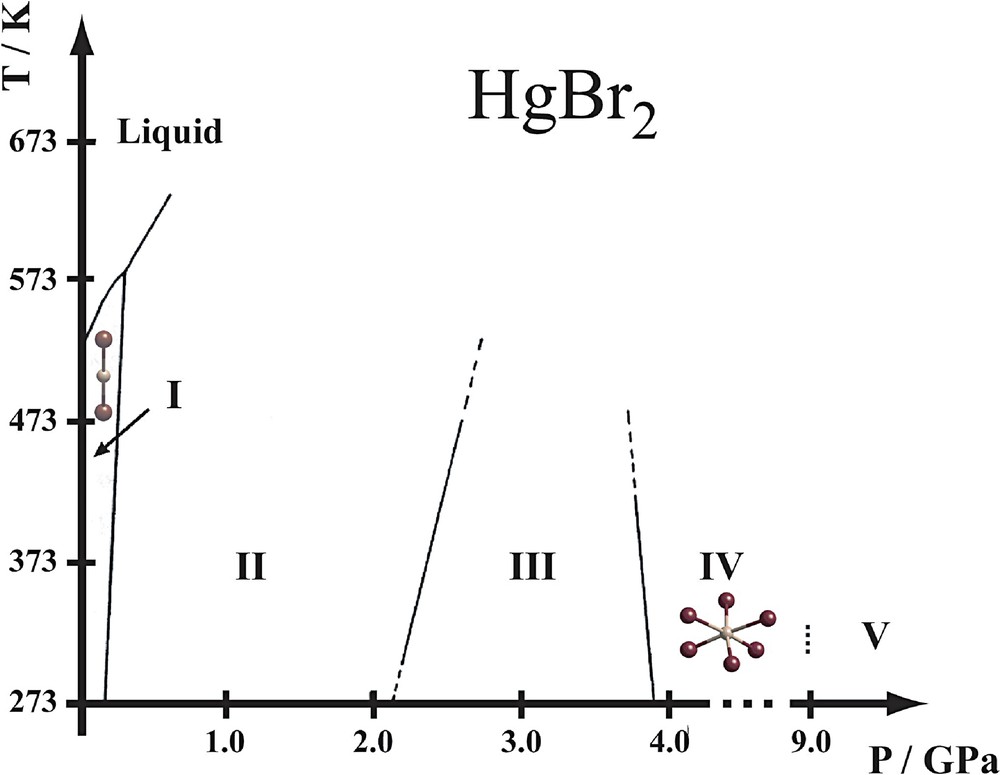
Phase diagram of HgBr2. The phase boundaries between phases I to IV have been established by Raman spectroscopy [26]. The IV–V phase transition has been identified by X-ray diffraction [27].
3.2 Phase transition sequence under pressure
The I–II transition was first detected by dilatometry. It is accompanied by a volume change similar to the one observed for the II–III and III–IV transitions [25]. However, its existence was not conclusively confirmed by Raman spectroscopy [26] or by X-ray diffraction [27]. Phase III displays a more complicated diffraction pattern than phase I, additional powder lines suggesting a lower, possibly monoclinic symmetry. Attempts to index the pattern were unsuccessful. In addition, the corresponding Raman spectrum shows an inferior signal-to-noise ratio compared to the spectra from I, II and IV [26]. These difficulties might indicate the formation of a strongly disordered state within the stability range of phase III. The III–IV transition has a destructive character and leads to a well-defined powder pattern of a trigonal phase IV, the structure of which is a variant of the CdI2-type. It is built from layers of edge-sharing octahedra that are stacked by Br···Br contacts. In contrast to the CdI2-type structure, some of the coordinated metal atoms are displaced along c from the centroids of the octahedra, leading to three distinct mercury sites (Fig. 5). One of the three Hg atoms adopts a trigonal-pyramidal coordination with three nearest neighbours, whereas the other two show an approximately octahedral coordination with six bonds of roughly the same length [27]. The structure of phase IV may be described as a commensurate modulation of the CdI2 structure.
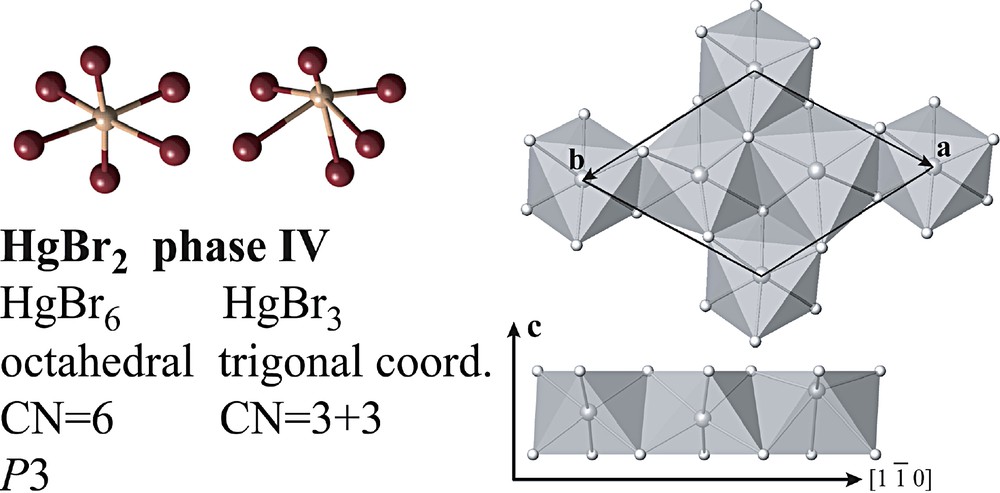
Structure of the trigonal phase IV of HgBr2 at 5.4 GPa showing layers of edge-shared octahedra. Note the alternation of the three mercury sites along the [1 0] direction.
4 Mercuric chloride
4.1 Structure of HgCl2 at ambient conditions
At ambient conditions, the structure of HgCl2 (phase I) is molecular with straight Cl–Hg–Cl molecules [28] that form herringbone layers in the mirror plane of the space group Pnma (Fig. 7). This structure is not related to HgI2-yellowHT and ambient HgBr2. The coordination of Hg is 2 + 6 (rather than 2 + 4) forming a distorted rhombohedron. The smallest distance between chlorine atoms of adjacent molecules is less than the sum of the van der Waals radii of Cl suggesting attractive Cl···Cl interactions. The lattice parameters of phase I may be expressed as an orthorhombic distortion of a cubic closest packing of chlorine atoms (Table 1).
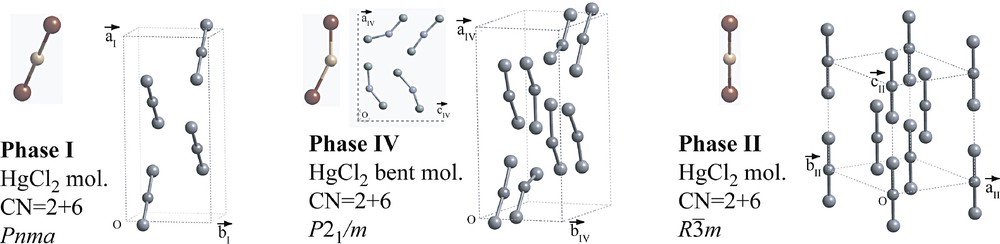
Structures of the molecular phases of HgCl2, phase I at ambient conditions, phases IV and II at high pressure. In phase IV, the molecules occupy the a/c mirror plane.
4.2 Phase diagram
Upon increasing temperatures at ambient pressure, the lattice constants show a decrease of the orthorhombic distortions of phase I, followed by a transition to a cubic phase V at 572 K, three degrees below the melting temperature [29]. The structure of phase V has not been investigated in detail; one might consider Hg to be disordered over, or diffusing between, voids of the cubic closest packing of Cl atoms.
Infrared, Raman [30] and NQR [31] spectroscopy revealed the existence of two high-pressure phases between 0 and 2.5 GPa at room temperature (Fig. 6). The structures of these two high-pressure phases have recently been determined from X-ray powder diffraction with synchrotron radiation [29]. At ambient temperature, HgCl2 undergoes a continuous transition to a monoclinic phase IV at 0.7 GPa. The structure of this phase is a slight monoclinic distortion of the orthorhombic phase I, β = 91.01(5)° at 1.7 GPa, whereby the mirror plane is preserved. There are four symmetry independent Cl–Hg–Cl molecules placed on the mirror that are now bent (Fig. 7). Phase IV is stable up to 2.1 GPa, at which pressure a first-order transition occurs to a rhombohedral Rm phase II showing straight molecules. Phase II is stable up to 6.0 GPa, at which pressure new powder lines appear in addition to those of the Rm phase. These lines have been attributed to phase III. Surprisingly, phases II and III coexist at least up to 20 GPa.
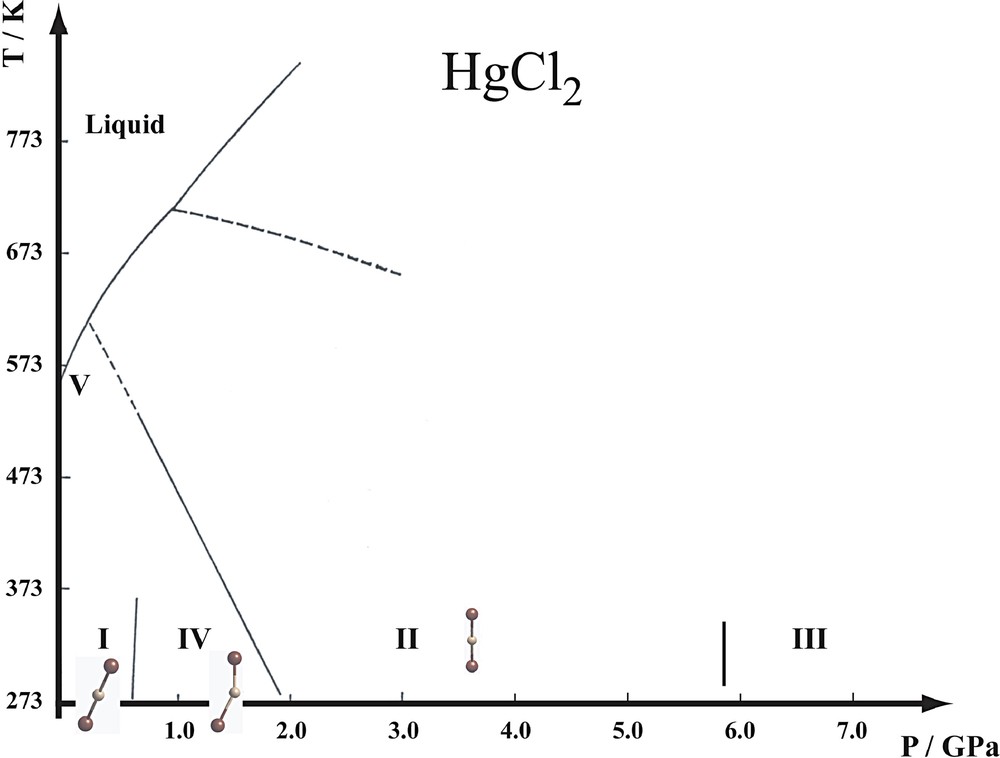
Phase diagram of HgCl2. The phase boundaries have been established by Raman spectroscopy, adapted from [30]. The nomenclature of the phases adopted in [30] has been retained.
5 Mercuric fluoride, structure of HgF2 at ambient conditions
At ambient conditions, HgF2 adopts the cubic CaF2-type structure [32] consisting of corner-sharing HgF8 cubes (Table 1, Fig. 8). So far, no information has been published on the phase diagram. Our attempt [29] to investigate the behaviour of this compound under pressure failed due to the fact that all available samples were hydrated.
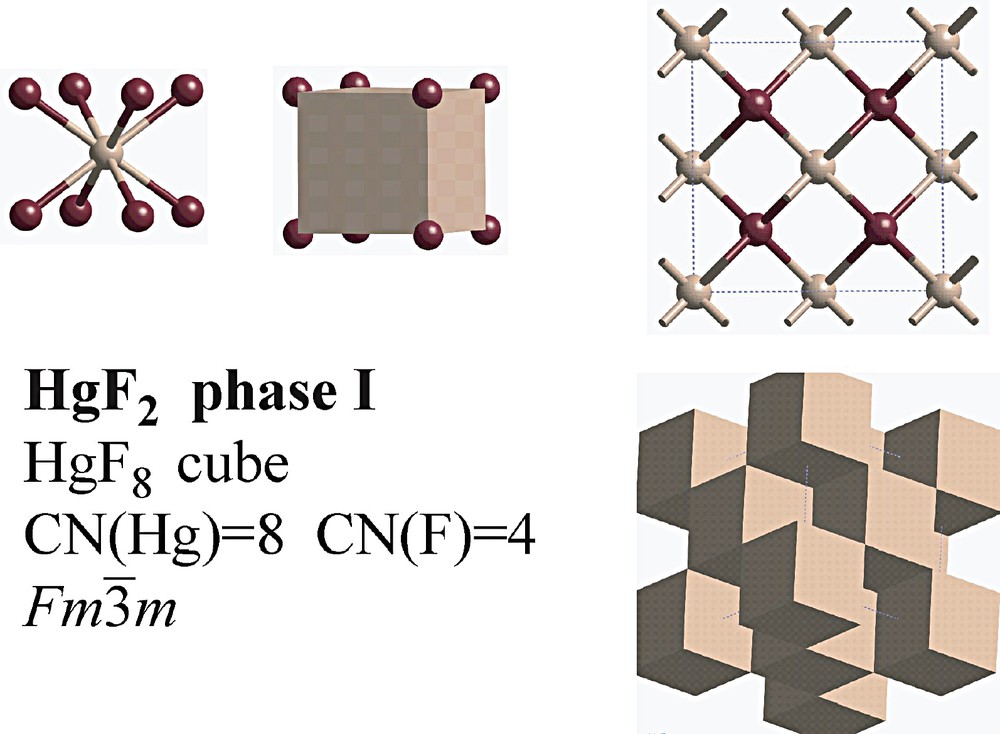
Structure of the fluorite-type phase of HgF2 at ambient conditions [32].
6 Discussion
The results presented here show that mercury(II) does not have a single preferred coordination with non-metallic elements. The numerous known structures of its four halides HgX2 show coordination numbers (CN) between two and eight. Coordinations of Hg include CN = 2 + 4 or 2 + 6 for linear or bent X–Hg–X molecules, tetrahedral CN = 4, trigonal pyramidal CN = 3 + 3 and octahedral CN = 6, and cubic CN = 8. HgI2 adopts many of these structures. At low pressures and temperatures, the coordination is tetrahedral, with CN = 4 and nearly perfect ccp packing of the iodine atoms. In this packing, Hg appears to be able to move between tetrahedral voids. High temperature and pressure stabilizes molecular structures with CN = 2 + 4 and bent I–Hg–I molecules. Above 7.7 GPa, the metric is hexagonal, but the lattice constants do not seem to correspond to a framework structure related to e.g. the CdI2 type. HgBr2 does not form tetrahedral structures. It favours molecular structures with CN = 2 + 4 in the low-pressure region, while pressures above 4.0 GPa stabilize pyramidal and octahedral coordinations. Two uncharacterized phases occur at intermediate pressures. HgCl2 forms only straight or bent molecular structures, but with a distorted rhombohedral coordination CN = 2 + 6. No information is available on the phase diagram of HgF2; the cubic phase I might be stable at high pressure. This is the only structure that could be interpreted as ionic.
It is interesting to compare these results with the halides of the other group IIB elements Zn and Cd [33]. These compounds do not form molecular structures. ZnF2 adopts the rutile structure, and the other halides form tetrahedral structures with closest-packed anions [33–37]. We recall that there are six simple tetrahedral structures with a ccp substructure of I. Four of these are realized by HgI2 red and orange, while ZnCl2 forms a fifth simple structure not (yet) found for HgI2, which is probably the most ionic one since it shows the most homogeneous cation distribution. The structure of red HgI2 has also been reported for ZnCl2. Interestingly, the supertetrahedral D-structure is the stable form of ZnI2, and also a metastable form of HgI2. CdF2 crystallizes with the CaF2 fluorite-type structure, as does HgF2. The other Cd halides form the well-known octahedral layer structures of which phase IV of HgBr2 is a variant. We may conclude that the group IIB difluorides follow the rules for ionic structures, CN increasing from 6 to 8 with increasing cation size. Indeed, the observed framework structures follow the classical ionic radius-ratio rule [33] surprisingly well: coordination numbers ought to be 4 for ZnCl2, ZnBr2, ZnI2 (R = rcation/ranion < 0.41), 6 for ZnF2, CdCl2, CdBr2, CdI2 (0.41 < R < 0.73) and 8 for CdF2, HgF2 (R > 0.73). However, the other Pauling rules for ionic structures are hardly obeyed, except for the fluorides and ZnCl2, since the cations do not adopt the most homogeneous distributions over the available voids of the anion packings and octahedra share too many edges. CN = 6 would also be predicted for HgCl2, HgBr2 and HgI2 which in reality form molecular and tetrahedral structures (with the exception of phase IV of HgBr2). Even though it is not surprising that HgI2 in particular is far from being ionic, we regret that ab initio quantum mechanical calculations are not yet available to elucidate the preferred bonding modes of these heavy atoms.
Acknowledgements
This work has been supported by the Swiss National Science Foundation.