1 Introduction
Since the first reported inclusion complex of C60 by γ-cyclodextrin [1], interest in constructing fullerene-containing supramolecules has continued. Addition of cyclotriveratrylene (CTV) to a toluene solution of C60 resulted in the formation of black crystals of CTV–C60 complexes [2]. The host–guest interaction of C60 with polybenzyl ether dendrimer functionalized CTV derivatives has been investigated by Nierengarten et al. [3]. Among the various useful hosts for fullerenes, calixarenes have been extensively investigated since the pioneering work performed by Atwood et al. [4] to purify C60 from carbon soot by selective complexation with p-tert-butylcalix[8]arene. In the calixarene family, host molecules including calix[3]-, calix[4]-, calix[5]-, calix[6]-, and calix[8]arenes have been found to form complexes with C60 and/or C70 [5–7]. In 1997, Ikeda et al. [8] studied solution complexes formed between C60 and calixarenes and found that not all calixarenes can form ball and socket structures with fullerenes. Fullerenes can also interact with porphyrins and metalloporphyrins through an interaction between a curved surface and a flat surface. This non-covalent recognition element was first confirmed by a crystal structure of a covalently linked tetraphenylporphyrin–C60 dyad [9]. Following that, a series of metalloporphyrin–C60 supramolecules have been systematically studied [10].
Recent research revealed that organized assemblies made of fullerenes and functionalized fullerenes exhibit potentially useful characteristics such as photovoltaic responses, charge transport, nonlinear optical properties, and biological activity [11–13]. A variety of methods have been developed to obtain fullerene-based ultra-thin films. Among these, self-assembled monolayers (SAMs) of C60-based molecules on solid substrates (Pt or Au) are one of the most used techniques for constructing highly ordered and definable thin films. Since the pioneering work of Shi et al. [14] to form well-defined SAMs of a C60 thiol derivative on gold surfaces, many reports have been published concerning C60 derivative SAMs by using donor-fullerene dyads and triads and these have been systematically used to build photovoltaic devices [15]. An ordered SAM of a C60–anthrylphenylacetylene hybrid on a gold surface exhibited interesting electronic properties [16]. Recently, the first four-electron reduction of a C60–metal cluster SAM was observed [17]. In 1996, our group successfully assembled a fullerene–crown ether derivative on gold surfaces through the non-covalent interaction between surface-attached ammonium groups and the crown ether moiety [18]. We have also reported SAMs of a fullerene derivative via the adsorption of 1,10-phenathrolnyl nitrogens on gold surfaces [19].
In all of the methods described above to prepare fullerene based thin-film materials, covalent functionalization of C60 was required, which partially destroys the electronic π-delocalization of the molecule due to the introduction of the adduct. In addition, C60 and its derivatives have a high aggregation tendency which affects its molecular electronic properties. To minimize both problems, Shinkai et al. accomplished a non-covalent incorporation of isolated C60 molecules by complexation with homooxacalix[3]arenes on surfaces, combining electrostatic binding and π-stacking [20]. The photocurrent flow observed for such photoactive ITO electrodes exhibited a very high quantum yield (21%) upon irradiation. Considering the obvious advantages of non-covalent immobilization of C60 on solid substrates over the conventional methods, we recently prepared a series of calix[n]arene, porphyrin and CTV derivatives with surface anchoring groups and investigated their abilities to supramolecularly incorporate C60 on gold electrode surfaces. Herein, we summarize our recent results in this area.
2 Results and discussion
2.1 Calix[n]arene receptors
The first set of receptors that we synthesized was a series of thioctic ester derivatives of p-tert-butylcalix[4]arene and p-tert-butylcalix[4]crown-6, as shown in Scheme 1. Three conformational isomers of bis-thioctic ester derivatives of p-tert-butylcalix[4]crown-6, cone isomer 1, 1,3-alternate isomer 2 and partial-cone isomer 3 were prepared [21]. For comparison, one bis-thioctic ester derivative of p-tert-butylcalix[4]arene 4 was also synthesized. All the target compounds were characterized by 1H and 13C-NMR, IR and MS spectroscopies and elemental analysis. Different conformations of these calix[4]arene derivatives were assigned on the basis of their 1H and 13C-NMR spectra.
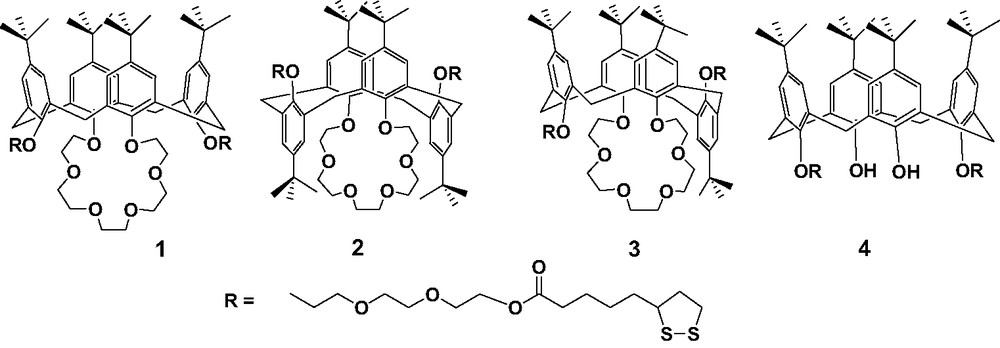
Calix[4]arene thioctic ester derivatives 1–4.
SAMs of 1–4 were formed on gold surfaces by dipping freshly prepared gold beads or gold film on silica microslides into deaerated acetonitrile solutions of the appropriate thioctic ester derivatives. Theses SAMs were characterized well by contact-angle measurements, reflection–absorption infrared spectroscopy (RAIRS), electrochemical blocking experiments and impedance spectroscopy [21a]. The water contact angles were observed to be 97 ± 2°, 83 ± 2°, 85 ± 2°, and 87 ± 2° for SAMs of 1–4, respectively, in comparison with 44 ± 2° for bare gold. These data indicate that hydrophobic films were formed on these gold surfaces due to the bulky p-tert-butyl groups and the benzene rings on the calix[4]arene frame. By comparing the transmission IR spectrum of 2 and the RAIRS spectrum of the corresponding monolayer, variations of the stretching modes of the carbonyl groups and C-O-C groups from the anchoring chains were observed, indicating that the orientation of the molecules is fixed after the monolayer is formed on gold surfaces.
SAMs of 1–4 were characterized by observing their blocking effect on the cyclic voltammetry (CV) responses of a Ru(NH3)63+/2+ redox probe. The typical CV response for the Ru(NH3)63+/2+ redox couple at a gold electrode modified with 4 is shown in Fig. 1. Some variations were observed for the different SAM modified gold electrodes but all showed reasonably effective blocking effects. A large cathodic current was observed, indicating that a relatively fast electron transfer process is still taking place at the interface. This is ascribed to loosely packed monolayers and/or good electron permeability through the monolayer. Further characterization of the SAMs was performed by electrochemical impedance spectroscopy. The typical Nyquist plot of SAM modified gold electrodes by 1–4 exhibit both a semicircle and a straight line. The diameter of the semicircle represents the charge transfer resistance caused by the blocking of electron transfer by the monolayers formed on the electrodes and the linear portion represents a diffusion-controlled process. In contrast, the Nyquist plot obtained on bare gold electrodes is simply a straight line (the Warburg impedance), because the redox reactions are very fast on bare gold surfaces and are controlled exclusively by diffusion processes.
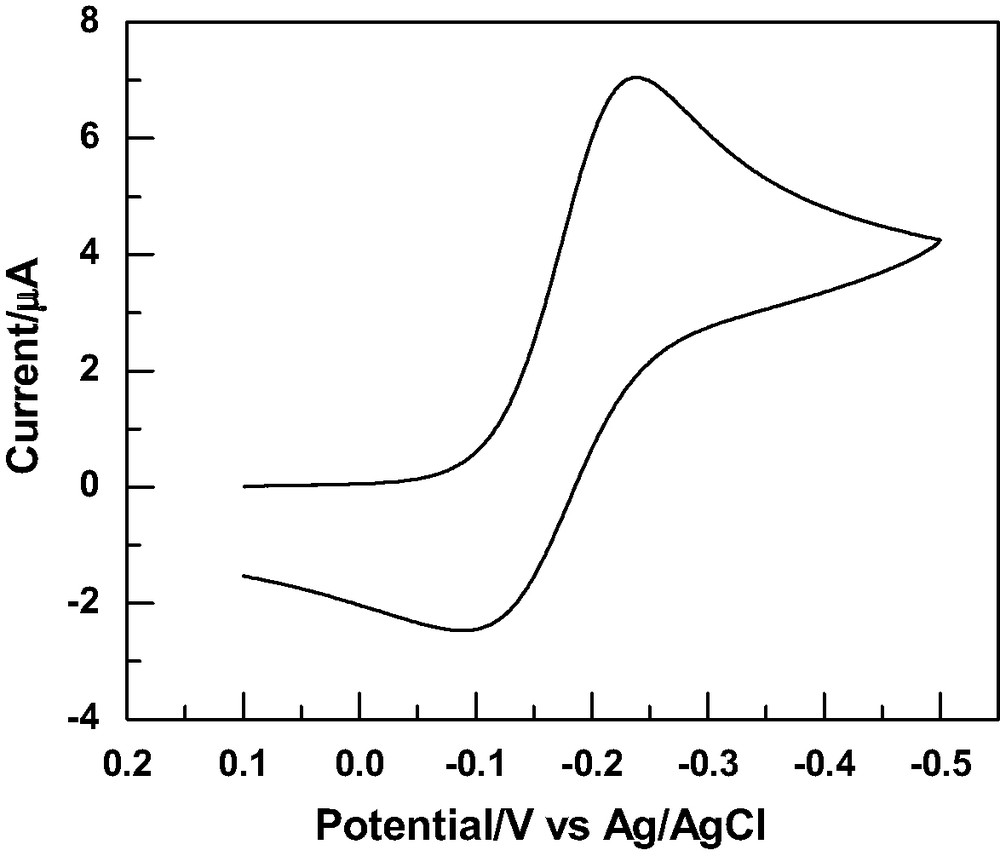
Cyclic voltammogram of Ru(NH3)63+/2+ at a gold electrode modified with SAMs of 4 at a scan rate of 100 mV/s.
SAMs of 1–4 were then immersed in C60 solutions in 1,2-dichlorobenzene in an attempt to immobilize C60 via supramolecular interactions. No redox response of the fullerene was observed in any of the cases studied, indicating that these SAMs could not bind C60 effectively. However, these SAM-modified gold electrodes exhibited interesting metal cation recognition properties. Impedance spectroscopy was used to study the interfacial ion recognition process. The complex impedance response of SAMs of 1 exhibited very little change of the charge transfer resistance when exposed to Cs+, suggesting that compound 1 cannot bind Cs+ significantly [21b]. The SAM modified gold electrode with 2 showed a completely different response towards Cs+ when compared to the SAMs of cone-isomer 1. Addition of Cs+ to the electrolyte gave rise to a significant increase in the value of Rct. This indicates complexation of Cs+ at the interface and the repulsion of the positively charged redox probe, Ru(NH3)63+/2+ [21b]. The selectivity for Cs+ was dependent on the size fit between the crown ether and the ion, as well as a cation-π interaction between the Cs+ and the two rotated aromatic rings. The conformation of the calix(4)arenes plays a key role in the selectivity of calix[4]crown-6 derivatives for the metal cations. In comparison with derivative 1, the isomer 2 exhibits a much more favorable conformation to bind Cs+ by coupling the complexation ability of the crown ether to the metal cations with the interaction between the aromatic centers and Cs+. This was the first report of the self-assembly of different conformers of p-tert-butylcalix[4]crown-6 derivatives on gold electrodes and their Cs+ recognition controlled by conformational differences. Only the SAMs of 2, in the 1,3-alternate conformation, were able to bind with Cs+ to some extent, while other SAMs showed no recognition ability for alkali metal cations. However, all SAMs of 1–4 showed binding properties with alkaline earth metal cations such as Ca2+ and Ba2+, as confirmed by CV blocking experiments and impedance spectroscopy [21a].
Considering that the cavity of calix[4]arene derivatives may be too small to bind C60 effectively once they were assembled on the gold surfaces, we further synthesized calix[6]crown-4 derivative 5 and calix[8]arene thioctic ester derivative 6, as shown in Scheme 2. The 1H-NMR spectrum suggested a 1,4-bridged cone conformation for 5. SAMs of 5 were characterized by CV blocking experiments and impedance spectroscopy. Like the calix[4]arene thioctic ester derivatives, SAMs of calix[6]crown-4 derivative 5 [22] were not effective for the non-covalent immobilization of C60 on gold surfaces, since no redox response for the fullerene was observed except the typical one for physically adsorbed C60. In contrast, SAMs of the calix[8]arene derivative 6, possessing a bigger cavity, are able to incorporate C60 on gold surfaces after immersion in solutions of C60 [23]. Two reversible redox waves were observed for SAMs of 6 after the incubation in solutions of C60, which correspond to the first and second reduction processes of C60. This confirms the incorporation of C60 on gold surfaces. The electrochemical behavior is in agreement with that obtained for the non-covalent fullerene immobilization by CTV derivatives [24]. The peaks are broad and the first reduction process consists of two overlapped peaks, suggesting the presence of different binding states of C60.
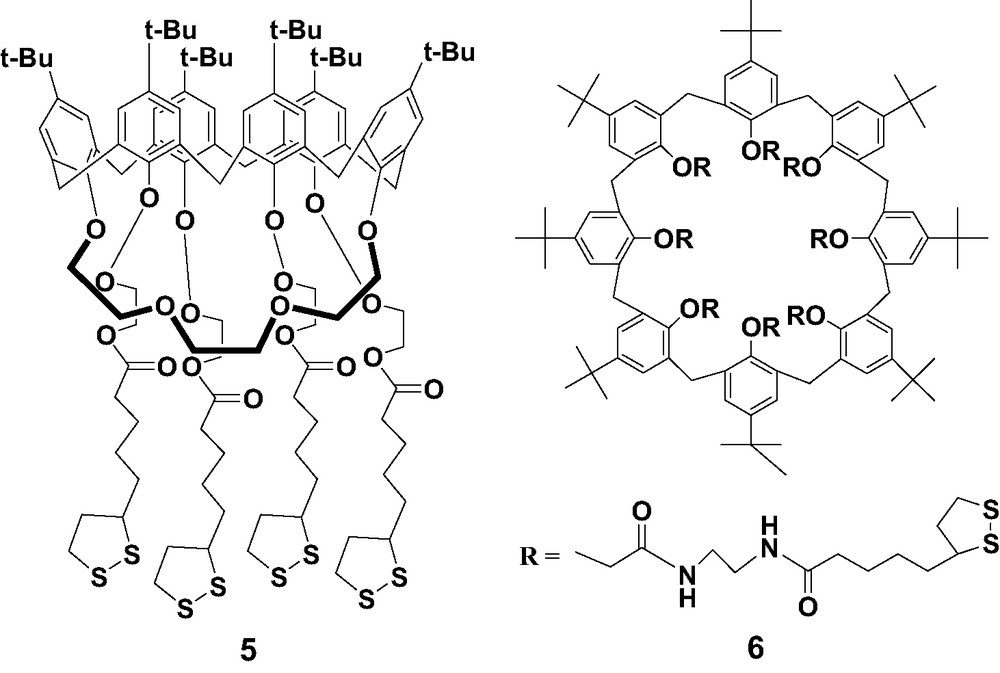
Calix[n]arene (n = 6, 8) thioctic ester derivatives 5 and 6.
Further investigation of SAMs of 5, however, revealed their remarkably sensitive response for anilinium [22]. Fig. 2 shows a comparison of the impedance response of SAMs of 5 to alkylammonium and anilinium chloride. Addition of 80 mM of any alkylammonium chloride lead to an Rct value of 52.6 K Ω at its maximum limit. However, addition of only 2 mM of anilinium chloride to the electrolyte gave an Rct value of 145.6 kΩ, showing that anilinium chloride interacts with the monolayer much more strongly than the alkylammonium cations. The remarkably efficient recognition of anilinium chloride by SAMs of 5 is probably related to a good size correspondence between the calix[6]crown-4 cavity and the guest aniline. The binding could also be affected by other interaction forces such as the cation-π interactions, hydrophobic interactions, CH3–π and π–π stacking between anilinium and the host monolayer. The binding affinity of 5 towards other biogenic aromatic amines such as dopamine was also investigated. Both CV blocking experiments and impedance spectroscopy showed that SAMs of 5 exhibit much better recognition for dopamine than for the alkylammonium salts, making it a potential candidate for sensing dopamine and its analogues.
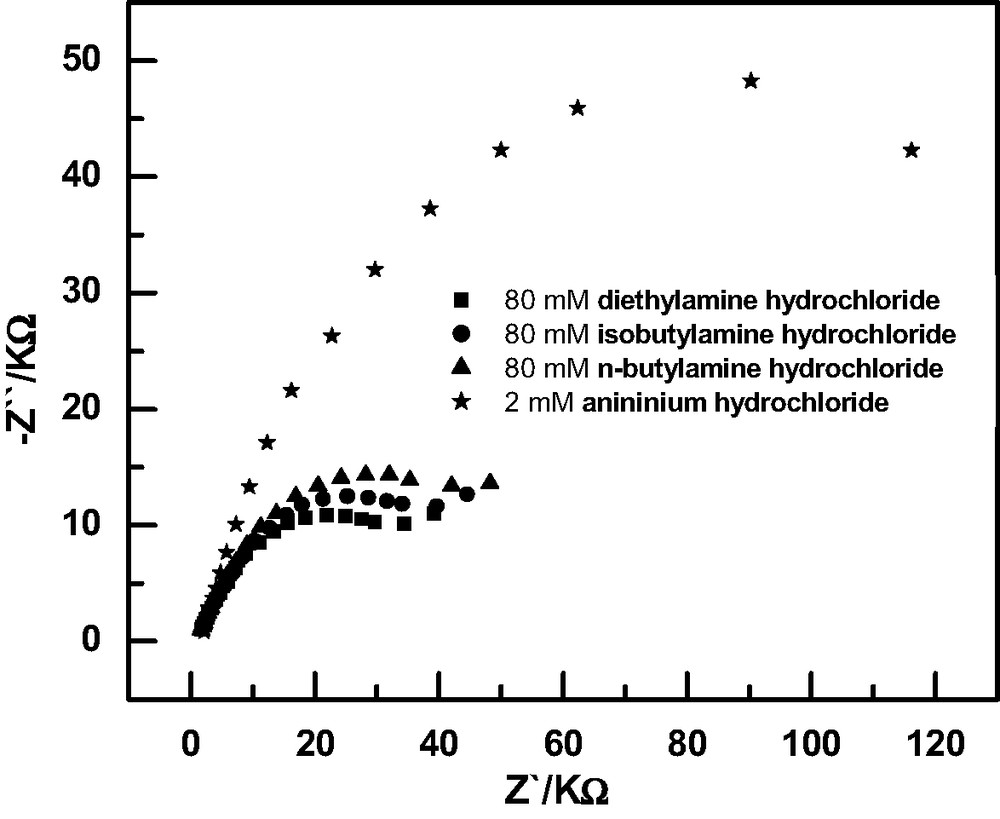
Impedance response of Ru(NH3)63+/2+ at the monolayer modified gold electrode with 5 in the presence of 80 mM of alkylamine hydrochloride and 2 mM of aniline hydrochloride.
2.2 CTV-based C60 receptors
In addition to calix[n]arene derivatives, we also prepared two cyclotriveratrylene (CTV) derivatives with surface anchoring groups, since CTVs are also known to be good fullerene hosts [2,3]. The structures of CTV derivatives 7 and 8 are shown in Scheme 3. The complexation of 7 and 8 with C60 was first investigated in solution by observing the changes of the absorption spectrum of C60 in toluene upon addition of increasing concentrations of the receptors [24]. The spectral changes are more pronounced for receptor 8, while the spectra of C60 in the presence of 7 showed no appreciable changes in the absorbance even after the addition of 40 equivalents, indicating a stronger interaction with 8 than with 7.
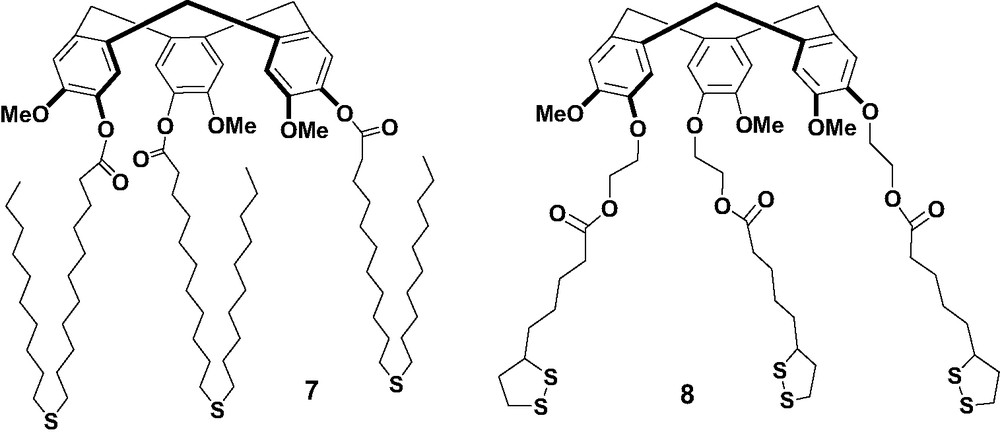
CTV derivatives 7 and 8.
Self-assembled monolayers of 7 and 8 were formed on gold surfaces and characterized by CV blocking experiments, impedance spectroscopy and electrochemical reductive desorption. Desorption experiments were conducted by dipping the SAM modified gold beads into thoroughly deoxygenated 0.5 M KOH. The cyclic voltammograms recorded on gold electrodes covered with SAMs of 7 or 8 show irreversible waves at –1.0 V vs. Ag/AgCl, which are due to the reductive desorption of the surface attached thiolates. By integrating the current under the cathodic wave, estimated surface coverages of 3.26 × 10−10 and 4.47 × 10−10 mol/cm2 were obtained for SAMs of 7 and 8, respectively. The data obtained by CV, impedance and desorption experiments indicate that SAMs of 8 are more compact than those of 7. This indicated that the dithiolane moiety of thioctic acid interacts stronger with the surface than thioether groups.
Non-covalent immobilization of C60 on gold surfaces was obtained with SAMs of the two CTV derivatives [24]. SAMs of 8 can bind C60 after they are formed or incorporate C60 if it is present during SAM formation. Fig. 3 shows two well-defined reversible redox waves at E1/2 = –0.93 and –1.34 V versus Ag/Ag+, which correspond to the first and second reduction processes of C60, respectively, revealing the incorporation of C60 in the SAMs. The relatively broad peaks suggested the presence of different binding sites of C60. C60 can be encapsulated in the cavity of 8 or introduced into the space between the attached CTV molecules. Most interestingly, the color of the gold bead electrodes changed significantly from an original copper color to dark red upon successive scans. This might be attributed to charge transfer processes associated with complexation and to the rearrangement of the monolayer structure. This color change was reversible, but it required ultrasonication in CH2Cl2 for 30 min.
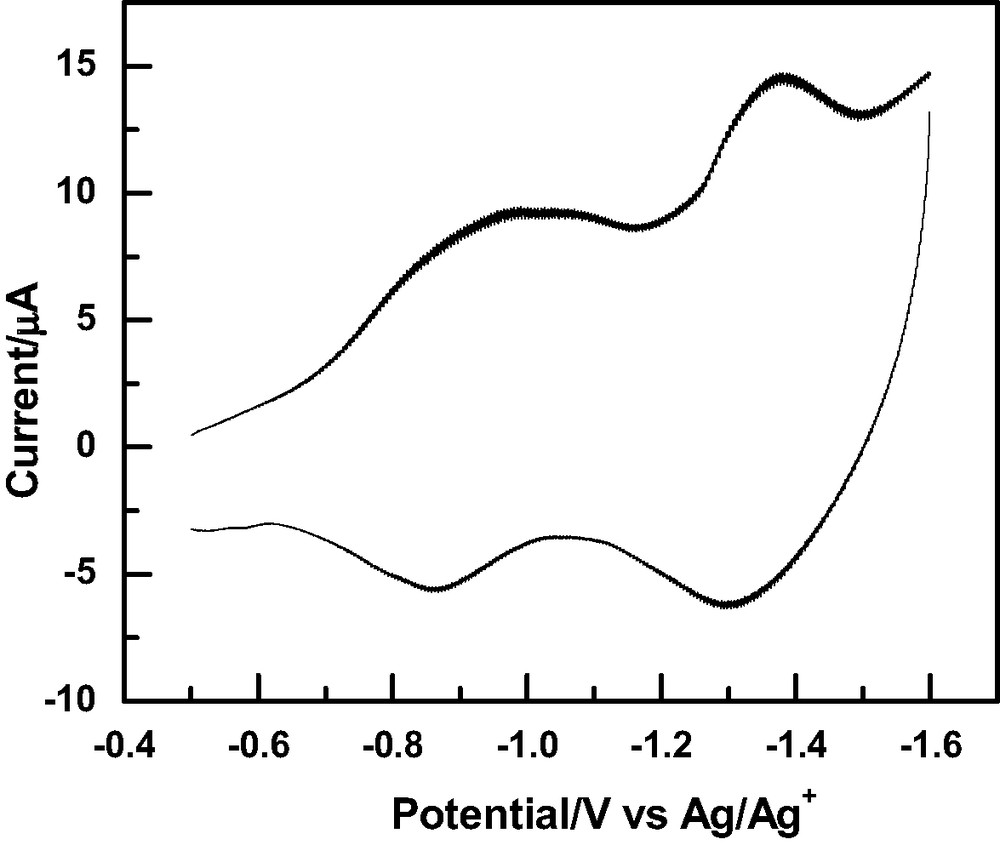
CV recorded in CH3CN of SAMs of 8. Supporting electrolyte: 0.1 M Bu4NPF6. Scan rate: 0.1 V/s.
Different from those of 8, SAMs of 7 could not incorporate C60 by the incubation of the monolayer in solutions of C60 since no electrochemical response for the fullerene was observed. The CV of SAMs of 7 after the incubation in solutions of C60 exhibited a typical electrochemical response of physically adsorbed C60. The long alkyl side chains probably blocked the incorporation of C60 in the CTV cavity. Experiments were then conducted to incorporate C60 during SAM formation of 7. After immersing the gold beads into a freshly prepared 1,2-dichlorobenzene solutions containing 7 and C60 for 2 days, still no faradaic response for C60 was observed. This indicates that the monolayers could not bind the fullerene, which is consistent with the UV titration experiments. However, if the mixture of 7 and C60 was kept for 2 weeks and SAMs were then grown from the resulting solution, two reversible waves for C60 were observed, suggesting that complexation between C60 and 7 is a very slow process. Further experiments are necessary to probe the nature and mechanism of this slow process.
2.3 Immobilized porphyrins as potential hosts for C60
As indicated in the introduction, fullerenes can also bind porphyrin derivatives via the so-called planar–convex interaction. Three porphyrin derivatives 9–11 (Scheme 4) were thus prepared. The CVs of compounds 9–11 in CH2Cl2 features two reversible one-electron reduction couples and the first reversible oxidation wave [25]. The second oxidations of these porphyrin compounds are not reversible. SAMs of compounds 9–11 were formed on gold surfaces by dipping gold bead electrodes into CH2Cl2 solutions of the target compounds. Two reduction processes at –1.57 and –1.95 V vs. Ag/Ag+ were observed for the gold electrodes modified with SAMs of 10. All peak potentials are proportional to the sweep rate, ranging from 0.1 to 0.8 V/s. This indicates a surface-confined behavior due to the immobilization of the electroactive porphyrin derivative on the surfaces. Like in the case of 10, SAMs of 9 and 11 exhibited very similar electrochemical behavior. These SAMs are very stable and their electrochemical response remains essentially the same after multiple scans.
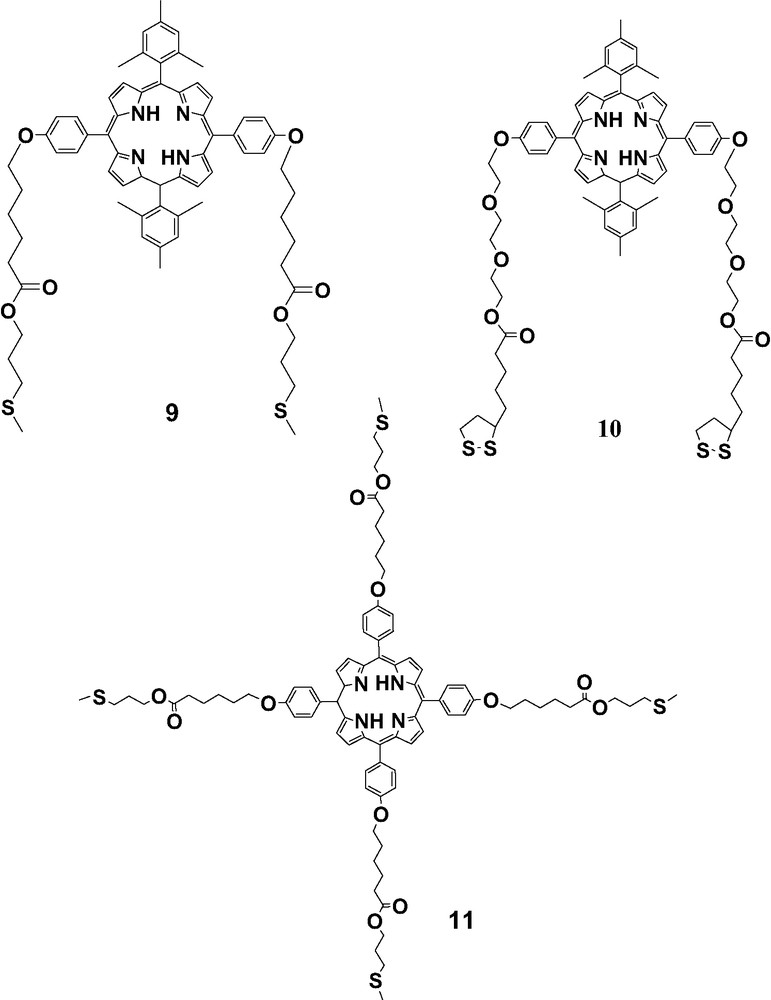
Porphyrin derivatives 9–11.
SAMs of porphyrin compounds 9–11 were immersed into 1,2-dichlorobenzene solutions of C60 to attempt non-covalent incorporation of the fullerene [25]. Fig. 4 shows the electrochemistry of SAMs of 10 after the incubation in 1,2-dichlorobenzene solutions of C60. In the first scan, four reduction peaks were observed at –0.94, –1.33, –1.56 and –1.93 V vs. Ag/Ag+, respectively. The first two reduction potentials are almost the same as those observed for C60 immobilized on gold surfaces by CTV derivatives, which correspond to the first two reduction processes of C60, thus confirming the incorporation of C60 on gold surfaces by SAMs of 10. The third and fourth redox waves reversible redox processes correspond to the first and second reduction processes of the porphyrin moiety. Compared to the redox responses of free SAMs of 10, the reduction peaks of the porphyrin after incorporation of C60 are broader and a shoulder peak at –1.67 V was observed, indicating the formation of C60–porphyrin complexes on the gold surfaces. The peak intensities of the first two reduction waves decreased upon successive scans and eventually only the redox responses for the porphyrin group were observed, suggesting that these fullerene–porphyrin complexes are not very stable.

CV recorded in CH3CN of SAMs of 10 after dipping into a solution of C60.
Similarly, SAMs of 9 were also used in an attempt to immobilize C60 on gold surfaces. The first scan of SAMs of 9 after the incubation in solutions of C60 exhibited a rather complex electrochemical response with several broad peaks, probably derived from the overlap of the reductions of porphyrin and C60 in different complexation modes. The successive scans changed the CV dramatically, revealing some structural rearrangement of the porphyrin–C60 complexes, and/or the injection of TBA+ during the assembly process in the SAMs. After several scans, four reduction peaks were observed. The first and second reductions correspond to the first two reduction processes of C60, indicative of the incorporation of C60 into the SAMs of 9. The third and fourth reductions are reversible redox waves, which are attributed to the first and second reduction processes of compound 9. SAMs of 11, however, were not effective at trapping C60 on gold surfaces in the same way. Electrochemical studies also show that such surface complexes of C60 are not stable upon successive reductive scans, probably due to a very weak binding interaction.
In summary, a variety of calix[n]arene, cyclotriveratrylene and porphyrin thioctic ester derivatives have been synthesized as potential surface hosts for C60. SAMs of these compounds were formed on gold surfaces and characterized by different methods. These stable SAMs were then used in an attempt to immobilize C60 on gold surfaces. Among the calix[n]arene derivatives, only SAMs of the calix[8]arene compound, with a relatively large cavity, can incorporate C60 onto the electrode surfaces. SAMs of calix[4]arene and calix[6]crown-4 derivatives exhibit, however, interesting metal cation and anilinium sensing properties. SAMs of CTV and porphyrin derivatives, except for porphyrin compound 11, were also reasonably effective for the non-covalent immobilization of [60]fullerene on gold surfaces.
Acknowledgements
Financial support from the National Science Foundation, (Grant No. CHE-0135786) is greatly appreciated.