1 Introduction
Due to their synthetic tailorability, high photostability, interesting electrochemical and photophysical properties, ruthenium(II) polypyridine complexes have played important roles in several areas of research, including solar energy conversion [1], solid-state light-emitting [2] or electroluminescent [3] devices, molecular biomimetics [4], biological immunosensors [5] or labels and probes [6], DNA conformation probes [7] or cleavage agents [8] and nonlinear optics [9] (NLO). Two-photon absorption (TPA) is a third-order NLO process involving the simultaneous absorption of two photons. After a short review of Ru(II) polypyridyl metal-to-ligand charge transfer (3MLCT) excited-state properties, some linear and nonlinear (TPA) photophysical applications relying on these properties will be presented and some perspectives will be drawn.
2 Excited-state lifetime engineering in Ru(II) polypyridyl complexes
Since the luminescence in ruthenium(II) polypyridyl complexes originates from a triplet metal-to-ligand charge transfer (3MLCT) excited state, it is possible to tune the related excited-state properties such as the luminescence wavelength, lifetime and quantum yield by tailoring the ligand structure and the rigidity of the complexes. The excitation into either a Franck-Condon 1IL charge transfer band, an appended chromphore via antenna effect [10] or the 1MLCT absorption band (see Fig. 1) is followed by a rapid intersystem crossing which leads to the population of accessible triplet states. Therefore, one of the initial steps of the relaxation is an ultrafast intersystem crossing (ISC) from the 1MLCT to the lowest-lying 3MLCT excited state in less than 20 fs. A plethora of such complexes have now been synthesized and studied by means of numerous spectroscopic techniques. They have been found to exhibit long luminescence lifetimes due to the triplet character of the emitting lowest 3MLCT excited state; a few nanoseconds to a few milliseconds (with, for example, terpyridine and 1,10-phenanthroline type ligands, respectively) are obtained for Ru(II) complexes [11–13]. A large number of relaxation channels contribute to the variation in the 3MLCT luminescence decay time at room temperature.
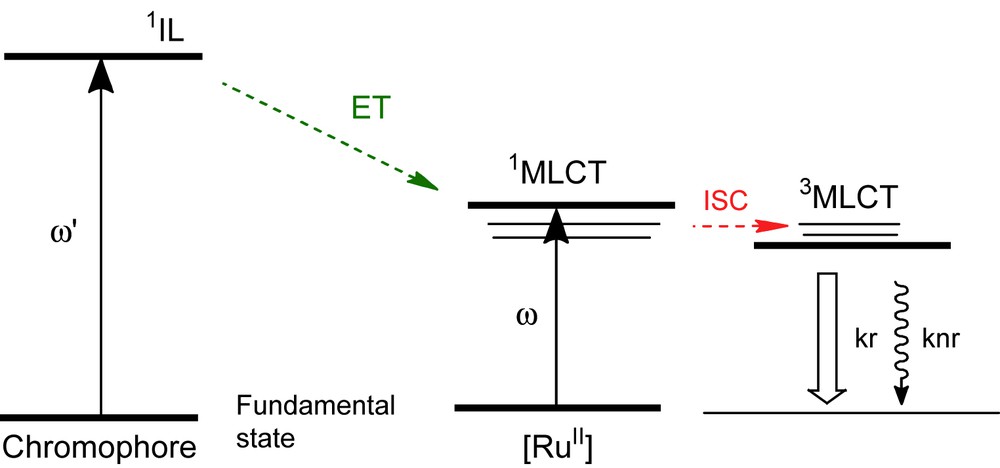
Simplified Jablonsky diagram related to energy transfer (ET), intersystem crossing (ISC) and radiative and non-radiative de-excitation processes (relative to kr and knr, respectively) within Ru(II) polypyridyl complexes.
Short lifetimes (particularly for compounds based on a tridentate polypyridine, whose prototype is [Ru(terpy)2]2+ with terpy = 2,2′:6′,2″-terpyridine) are due to deactivation via non-emissive short-lived metal-centered (3MC) states that get thermally populated from the 3MLCT state. Increasing the energy gap between the 3MLCT and the 3MC states may appear as an efficient means for enhancing the excited-state lifetime [14]. However, this approach often results in a decrease of the energy of the 3MLCT state, thus making these chromophores less useful for potential applications as photosensitizers. Recent strategies, involving polypyridine ligands with appended pyrenyl chromophores, have led to a dramatic increase of the 3MLCT room-temperature lifetime up to a few hundred microseconds [15]. A thermal triplet equilibrium has been suggested in this phenomenon [10,16]. The long-lived 3MLCT excited state of the Ru complex enables decay time measurements in biomedical applications [17] and allows one to free oneself from tissue autofluorescence (which typically decays within several nanoseconds, and thus does not interfere with the detection of the emission of the ruthenium complexes at longer times).
Consequently, these states can be used as probes and reporter molecules (in oxygen sensors for example), or even for the indirect characterization of dark excited states. Moreover, they are able to be involved in chemical reactions, for instance with ground-state dioxygen (3O2) in order to produce the cytotoxic singlet 1O2.
3 Two-photon absorption phenomena and applications
Upon irradiation by intense laser pulses, molecules and related molecular materials can absorb simultaneously two photons; this is a very attractive third-order nonlinear optical (NLO) process particularly because of the quadratic dependence of the two-photon absorption probability on the laser intensity (Fig. 2).
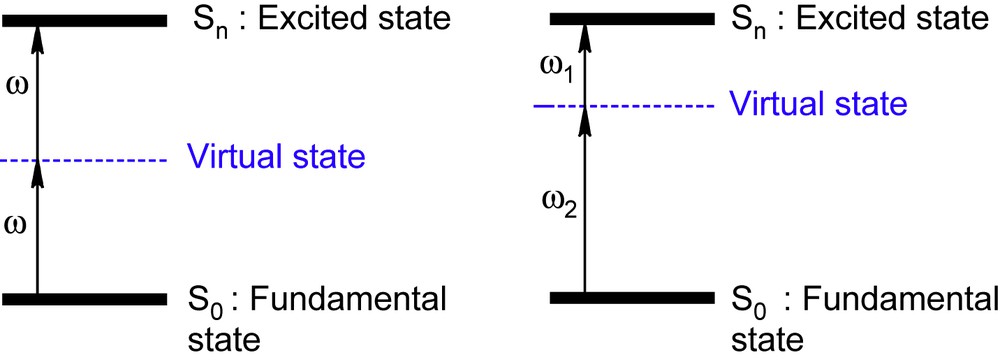
Two-photon absorption phenomena via excitation with (i) a unique laser at ω or (ii) two different lasers at ω1 and ω2; for non-centrosymmetric chromophores, Sn excited state may be the same with a one- or two-photon absorption.
The development of two-photon absorbing chromophores has become in the past decade [18] a growing research area whose progress is leading to a wide range of applications [19] such as three-dimensional optical data storage [20], 3D-fluorescence imaging [21], photochemical delivery of biological messenger [22], photodynamic cancer therapy [23], microfabrication [24] and Optical Power Limiting (OPL) [25]. TPA provides several advantages over currently used methods, because high-energy photophysical properties are activated by low-energy photons (in the near infrared) which allows greater material or tissue penetration than affordable with visible or UV-light; furthermore, the quadratic dependence on the intensity allows an enhanced control and a finer spatial resolution in various applications such as microfabrication and fluorescence imaging [26]. In this third-order nonlinear optics field, ruthenium(II) coordination and organometallic complexes [27] have received less interest than organic chromophores [28], in spite of undisputable advantages. The access via TPA to the 3MLCT state could therefore be exploited in fluorescence-based methods (two-photon excited fluorescence, TPEF) such as biological imaging or O2 sensing [29], as well as in processes involving excited-state re-absorption such as optical power limiting [30]. Concerning optical power limiting in nanosecond time scales, a further step has been reached by the occurrence, in the nonlinear absorption, of an excited-state absorption (ESA) process [31]. Actually, this ≪three-photon≫ (2 + 1) absorption process can be described as taking place in two steps during the nanosecond pulse duration; there is an instantaneous TPA followed by a very fast relaxation into the lowest-lying excited-state and then the ESA process takes place. Due to their long-lived excited states, high stability and inertia in solution, Ru(II) complexes are promising candidates in this area and have been recently used for such an application [32]. To the best of our knowledge, and as far as one is concerned with initial TPA phenomena in the near-infrared spectral range, only a rather limited number of examples have been reported in the literature about MLCT transitions by TPA at a single wavelength (750, 800 and 880 nm) for ruthenium(II) [33] and rhenium(I) [34] complexes; Z-scan experiments also gave rise to two-photon transitions spectra [35]. Recently and for the first time in ruthenium coordination complexes, detection of the MLCT band [36] led to a TPA enlargement (600–950 nm) with respect to that of related ligands.
4 Reactivity of the 3MLCT excited state of Ru(II) complexes toward O2
4.1 O2 luminescent sensors
As far as their use as luminescent dioxygen sensors in fields ranging from environmental to medical applications is concerned, Ru(II) polypyridyl complexes ([Ru(bpy)3]2+ type) have received the most interest [37] as compared to semi-conducting materials [38]. The detection principle is based on the luminescence quenching due to an energy transfer process between Ru 3MLCT excited state and the 3O2 ground state (see Fig. 3).

Evolution of the luminescence spectra of complex Ru(L1)3 in DMSO with concentration of dioxygen: Ru(L1)3–DMSO, Argon; Ru(L1)3–DMSO; Ru(L1)3–DMSO, O2 under argon, air and dioxygen, respectively.
The correlation between the O2 concentration ([O2]) and the luminescence intensity is ruled by the Stern–Volmer equation:
I0/I = 1 + Ksv[O2] |
For the engineering of dioxygen sensors and the development of other types of devices (humidity sensors [40] for example), Ru(II) complexes are usually incorporated into sol–gel materials [41,42], polymeric matrices [43], zeolites [44] or monolayer-based complex systems [45]. The advantages of these latter systems are to protect from pH polymerization or decomposition of the host matrix. All these examples, like atmospheric quality sensor materials recently reported by McGaughey [40], are based on linear interactions of light with matter (linear optics). Even though theoretical studies have claimed that a better precision can be achieved by performing nonlinear optical measurements [46], two-photon absorption has never been used for such detectors and this field of research is still to be explored.
4.2 Dioxygen detection in living cells and biological tissues
Dioxygen has an important role in our metabolism. Gaining a better understanding of this mechanism and more particularly its concentration in tissues, has important implications. This requires non-destructive analytical methods adapted to concentrations of the order of 10−4 mol L−1 in living cells or in vitro measurements. The method shown above, based on the luminescence quenching in Ru(II) complexes such as Ru(bipy)32+ and Ru(phen)32+, is without proved cytotoxicity in the conditions of the experiment [29a,47]. In this approach, the dioxygen concentration determination is based on luminescence decay measurements (3O2 decreasing 3MLCT lifetime through energy transfer) rather than through luminescence intensity as for conventional O2 sensors. The association of the FLIM (fluorescence lifetime imaging microscopy) imagery technique allows three-dimensional resolution of the dioxygen concentration in tissues to be obtained. Most often, linear optics is used; however, in order to achieve a better spatial resolution and to be able to irradiate the probe in the biological transparency spectral range (700–1000 nm in which the vibrational excitation of water can be neglected), TPA has also very recently been used with Pt(II) complexes [48].
4.3 Singlet dioxygen generation for photodynamic therapy (PDT) of cancer
The principle of photodynamic therapy (PDT) is based on energy transfer between triplet fundamental O2 and excited triplet state (see Fig. 3), generating cytotoxic 1O2 [49]. Contrary to biological applications such as dioxygen sensors and non-invasive oxygen measurements, the aim of the PDT is to use the phototoxicity of the singlet excited dioxygen to kill cancer cells. To the best of our knowledge, the use of ruthenium(II) complexes for this purpose has never been reported, despite the practical interest of long-lived 3MLCT excited state. Two-photon absorption phenomena may also be of great interest for PDT due to its higher precision and efficiency [50]. The candidate photosensitizers in this therapy [51] have to: (1) be without potential phototoxicity, (2) possess an amphiphilic character in order to go through the cell membranes, (3) be used in the biological transparence spectral range (700–1000 nm) and (4) possess high two-photon absorption cross-section.
First PDT agents are hematoporphyrine (HpD) derivatives and already used in the treatments of different cancers [52]; however, their low absorption in the near infrared leads to a low penetration in tissues (≤5 mm [53]). To overcome this problem, one must operate in the biological transparent spectral range (700–1000 nm), wherein TPA is of course of great interest. However, the currently used systems (porphyrins and tetrapyrroles) are poor two-photon absorbers [54] and only very few examples can be found in the literature. First attempts relied on the use of the meso-substituted and water-soluble porphyrins with two-photon absorption cross-section (σTPA) of the order 100 Göppert-Mayer (1 GM = 10−50 cm4 s photon−1 molecule−1) in the near infrared [55]. In order to increase σTPA values via antenna effect, these porphyrins have been incorporated into two-photon chromophore-based dendrimers. While aluminium-based porphyrins [56] gave the best results, those incorporating silver have proved not to be able to produce singlet oxygen. Studies of non-metallated porphyrins have also been reported [57]. A third strategy consists in the elaboration of self-assembly of zinc(II) metallated porphyrins connected by π-delocalized systems such as butadienes [58]. These systems exhibit both high TPA cross-sections (σTPA) of the order 7000–10 000 GM in the near-infrared spectral range [59] and efficiency for 1O2 generation. This high TPA cross-section is probably due to the delocalization of the π-electrons system between the two porphyrins through the bis-acetylene bridge. Water-soluble derivatives have recently been reported in the literature with σTPA = 8000 GM [60] and have revealed high toxicity for HeLa cancer cells. Despite their interesting photophysical properties, Ru(II) complexes have never been investigated in the literature in the field of photodynamic therapy associated with two-photon absorption.
Another strategy in PDT research is molecular functionalisation of biocompatible gold nanoparticles [61]; it presents two main advantages: (1) solubility in aqueous media and (2) the fine tuning of the optical properties through the control of the chromophore density. Studies concerning gold nanoparticles functionalized with ruthenium(II) complexes are reported in the literature [62]; nevertheless, most of them have been restricted to fundamental research concerning the optical properties.
5 Conclusion
This paper summarizes a large number of studies on the attractive properties of Ru(II) polypyridine complexes in their emissive 3MLCT state. The emphasis is put on the photoreactivity of the complexes towards triplet fundamental dioxygen (3O2) and its application in biology based on an initial TPA. The scope of this short review thus includes biological imagery and therapy (PDT). Optical power limiting is also briefly discussed.
For all these applications, the use of adequately substituted Ru(II) complexes or related molecular materials (sol–gel or gold nanoparticles) turns out to be an appealing route. Experiments are being conducted along this line in our research group, especially using PEG (polyethylenglycol group) substitution to obtain water-soluble complexes and achieve improved biocompatibility.
Acknowledgments
The authors would like to thank the CNRS, ENS-Lyon and the “Délégation générale pour l'Armement” (DGA) for financial support.