1 Introduction
Carbon dioxide is an available and abundant source that can be used as an initial substrate for the synthesis of a wide range of organic compounds [1]. But, the thermodynamic stability and relative kinetic inertness of CO2 limit the use of this molecule in chemical synthesis [2,3]. Therefore, to find new methods for CO2 activation is a challenge for organic chemists. In this regard, many catalytic species such as halostannanes [4], metal oxides [5], porphyrins [6], phthalocyanines [7], ionic liquids [8], and polyoxometalates [9] have been used for CO2 chemical activation. Moreover, the chemical activation of CO2 usually required high pressures [10] and high temperatures [11]. For example, CO2 has been used in chemical synthesis of cinnamic acid [12]. The strict conditions of the reaction such as low temperature (−50 °C) and usage of organometallic compounds limit this method for utilization [12]. In this regard, electrochemical reduction of CO2 is a suitable way of using this molecule in electrosynthesis of organic compounds such as carboxylic acids. However, high overvoltage is required for direct reduction of CO2 on the surface of different usual electrodes [13–16]. Therefore, for activation of CO2 in low overpotentials, it is necessary to introduce electron transfer mediators, such as transition metal complexes [17,18]. There are some articles on electrosynthesis of carboxylic acids by coupling reaction of CO2 with various unsaturated organic compounds [14,19–23]. In these reports, CO2 has been activated by different complexes [20,21], metal salts [22], organic compounds [19], and nickel or silver electrodes [14,23].
Cinnamic acid is used in certain pharmaceuticals and in the production of different esters in perfume industry [24]. The original ways to synthesize cinnamic acid are the Perkin reaction [25] and the Claisen condensation [26], which involve benzaldehyde as the initial monomer [27]. In this study, the [NiII(Me4-NO2Bzo[15]tetraeneN4)] complex was used for electrocatalytic reduction of CO2 in the acetonitrile (ACN) solvent. Then, the reduced form of CO2 (CO2 ˙−) was used for carboxylation of phenylacetylene to produce cinnamic acid. With respect to other catalysts, which have been previously reported in the literature, application of the Ni(II) complexes in carboxylation of unsaturated compounds has three advantages: (1) more increase in the reduction current indicating that the carboxylation of phenylacetylene is fast; (2) the potential shift of electrocatalytic reduction of CO2 to less negative values showing that the Ni(II) complex has an excellent electrocatalytic activity for CO2 reduction; and finally (3) the selectivity in the production of cinnamic acid.
2 Experimental section
2.1 Chemicals and apparatus
The [NiII(Me4-NO2Bzo[15]tetraeneN4)] complex, which has been denoted as Ni(II) complex from now on, was prepared as previously described [28,29]. The chemical structure of the Ni(II) complex is shown in Scheme 1. All the reagents were of analytical grade and were purchased from Merck Company and used without any further purification. Ar and CO2 had a purity of 99.995%. Cyclic voltammetry was performed using an EG&G PARSTAT 2273 equipped with a Power Suite software in a conventional three-electrode electrochemical cell containing a glassy carbon electrode (GCE) as the working electrode, a Pt wire as the counter electrode, and Ag/Ag+ (0.01 M AgNO3 in a 0.1 M tetrabutylammonium perchlorate (TBAP), ACN solution, freshly prepared) as the reference electrode. Controlled potential coulometry (CPC) was carried out using an SAMA 500 electroanalyzer system in a simple one-compartment cell fitted with a gas inlet and outlet with a graphite rod as the cathode, a magnesium plate (ca. 4 cm2) as the sacrificial anode, and Ag/Ag+ (0.01 M AgNO3 in 0.1 M TBAP, ACN solution) as the reference electrode. Fourier transform infrared (FT-IR spectrum analysis was performed using an EQUINOX55 spectrometer. 1H NMR and 13C NMR were measured using a DRX-400 (Bruker) spectrometer with dimethyl sulfoxide, (CD3)2SO, as a solvent in the presence of SiMe4 as an internal standard. All the measurements were performed at room temperature.
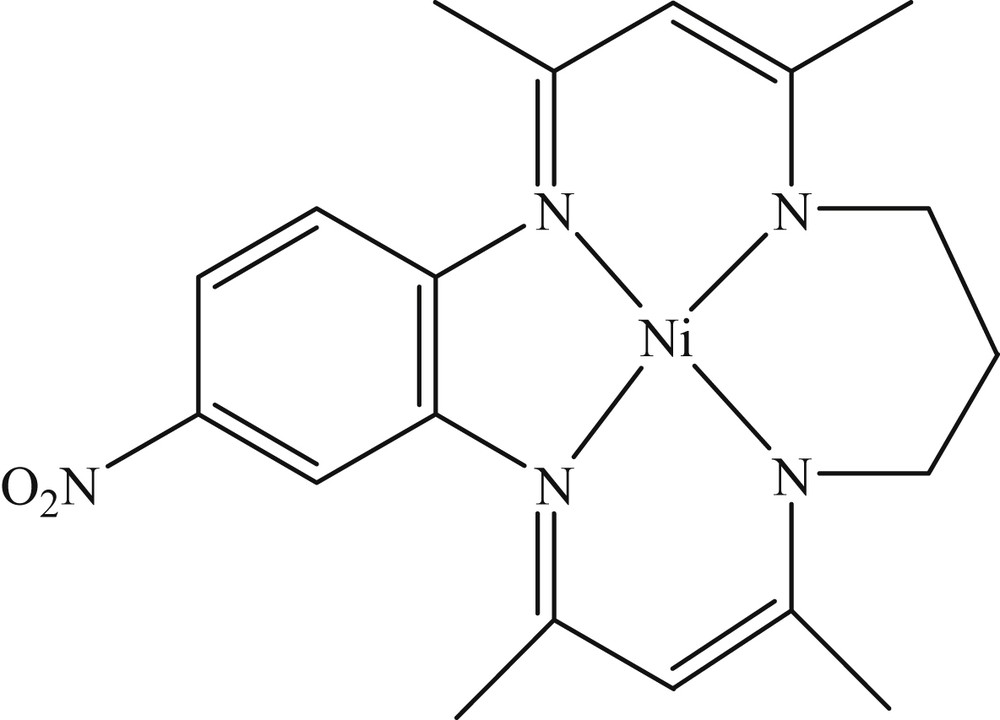
Chemical structure of the [NiII(Me4-NO2Bzo)2[15]tetraeneN4)] complex.
2.2 Electrolysis procedure
The electrolysis (CPC with constant potential of −1.72 V) was carried out in 40.0 mL ACN containing 0.1 M TBAP, 1.0 mmol of phenylacetylene, and 0.1 mM of the Ni(II) complex as an electrocatalyst. Before performing the experiment, the solution was bubbled with Ar gas for 10 min. During the electrolysis, CO2 was bubbled upon atmospheric pressure. The results showed that phenylacetylene conversion was about 100% after passing 2.3 F mol−1 of the starting compound at room temperature. At the end of the electrolysis, 5.0 mL of 0.01 M hydrochloric acid was added to the solution and it was stirred for 30 min at room temperature. Afterward, the solvent was completely removed, and the residue was dissolved in chloroform and filtered (4 × 25 mL). After evaporation of the chloroform, the residue was evaporated, and the product was separated by silica gel column. After separation, the yield of the main product was about 70%, which was characterized by FT-IR, 1H NMR, and 13C NMR. The spectral characteristics of the product were determined as follows: 1H NMR (DMSO, 400 MHz): 6.54 (d, 1H, J = 16), δ 7.62 (d, 1H, J = 16), δ 7.38–7.40 (multi, 3H), δ 7.66 (d, 2H, J = 3.9), δ 12.44 (s, 1H, CO2H) ppm; 13C NMR (DMSO, 100.6 MHz): δ 119.09, 128.12, 128.84, 130.16, 134.17, 143.95, 167.51 ppm; FT-IR: 1681 (CO), 1628 (CC aliphatic), 1095 (CO), 3445 (OH) (see Figs. S1–S3 of Supplementary Information).
3 Results and discussion
3.1 Electrochemistry of the Ni (II) complex in the presence of phenylacetylene and CO2
The electrochemical behavior of phenylacetylene and CO2 in the presence of the Ni(II) complex was studied in an ACN (0.1 M TBAP) solution and at room temperature using cyclic voltammetry method. Cyclic voltammogram (a) of Fig. 1 is related to the solution containing saturated CO2 at the potential range of −1.2 to −2.0 V. The voltammetric responses of 1.0 mM phenylacetylene alone and 1.0 mM phenylacetylene in the presence of CO2 are the same as that has been shown in voltammogram (a) of Fig. 1. As seen, no redox peaks are observed in this potential range. These observations demonstrate that the mixture of CO2 and phenylacetylene and also each one individually are not electroactive at this potential range at the GCE surface [29,30]. The cyclic voltammogram of the Ni(II) complex (Fig. 1, voltammogram b) shows a redox couple with a formal potential (E0′) of −1.63 V (redox couple I/II) versus the reference electrode. According to reaction (1) in Scheme 2, this redox couple corresponds to the reduction/oxidation process of the metal center [7,27,31]. The voltammogram (c) of Fig. 1 shows the response of the Ni(II) complex solution after subjecting to 1.0 mM phenylacetylene. The comparison of voltammograms (b) and (c) of Fig. 1 indicates that there is no interaction between the complex and phenylacetylene. The voltammogram (d) of Fig. 1 shows the response of the complex solution after bubbling of CO2 at the potential range of −1.2–−2.0 V. As it can be seen, when the complex solution is saturated with CO2, the cathodic current response increases dramatically (peak I) and the anodic peak current (peak II) corresponding to the complex is disappeared. This is a sign for mechanism and electrocatalytic activity of the complex toward CO2 reduction. As previously described, this catalytic effect corresponds to an electron transfer from reduced form of the complex, [Ni(I) complex], to CO2 and formation of CO2 ˙− (reaction (2) in Scheme 2) [27,30]. Also, after addition of CO2, the cathodic peak of the Ni(II) complex shifts about 100 mV to more negative potentials. This observation is due to the interaction of methine site of the Ni(II) complex with CO2 that is a reactive nucleophilic center [27,30,31]. Cyclic voltammogram (e) of Fig. 1 is related to the solution of the Ni(II) complex in the presence of CO2 after adding 1.0 mM phenylacetylene. A comparison between voltammograms (d) and (e) of Fig. 1 shows an enhancement in the catalytic reduction current and also 60 mV shift in potential to less negative values. These results demonstrate the interaction between phenylacetylene and electroactivated CO2, which is described in the following section.

Cyclic voltammograms of a GCE in an ACN solution containing (a) 0.1 M TBAP as the supporting electrolyte, (b) same as (a) in the presence of 1.0 mM Ni(II) complex, (c) same as (a) in the presence of 1.0 mM Ni(II) complex + 1.0 mM phenylacetylene, (d) same as (a) in the presence of 1.0 mM Ni(II) complex + CO2, and (e) same as (a) in the presence of 1.0 mM Ni(II) complex + CO2 + 1.0 mM phenylacetylene. Potential scan rate: 100 mV s−1.
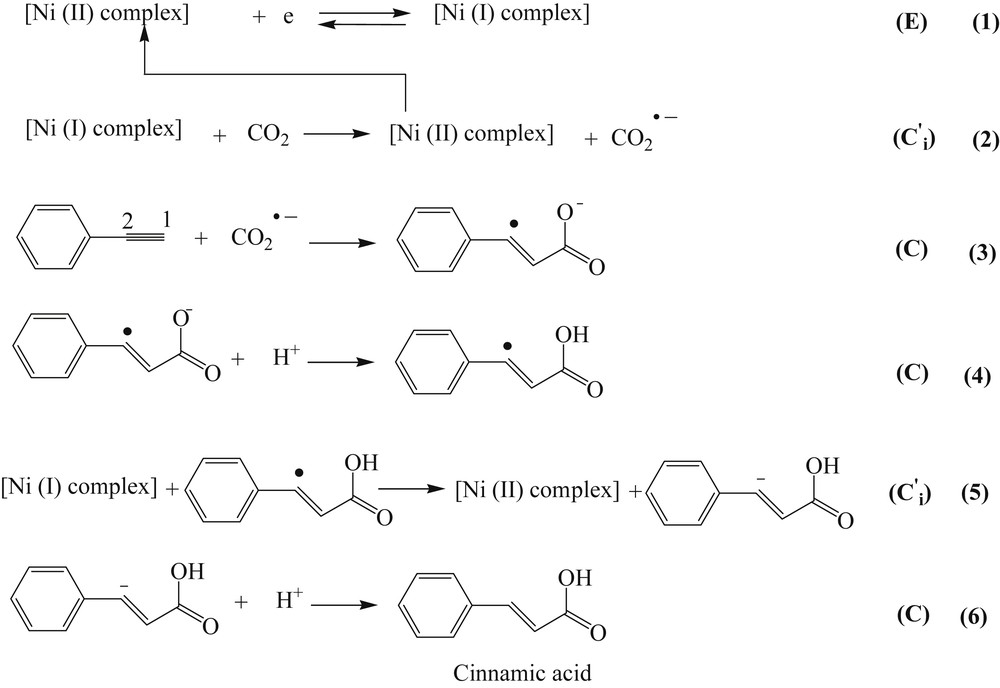
The mechanism for coupling reaction of phenylacetylene and electrocatalytic activated CO2, CO2 ˙−, in the presence of the Ni(II) complex and production of cinnamic acid.
3.2 Application of electrocatalytic activated CO2 for carboxylation of phenylacetylene
According to the literature, the product(s) of the coupling reaction of the phenylacetylene with CO2 depends on the reaction conditions. In the absence of any catalyst, 2-phenylsuccinic acid is formed from the phenylacetylene reaction with CO2 at a high overpotential [21]. It was reported this reaction required a high pressure at the nickel electrode surface [21]. Also, synthesis of 2-phenylsuccinic acid was reported as a result of the reaction of CO2 ˙− with phenylacetylene or reduced form of phenylacetylene with CO2 at high overpotentials [23,32]. The saturated dicarboxylic acids and tricarboxylic acids were produced in the presence of metal salts (CuI, FeCl3) [22]. Also, a mixture of monocarboxylic acids was produced by using nickel–bipyridine complex as a catalyst [21–33]. In this study, to find out whether the reaction between electrocatalytic activated CO2 and phenylacetylene leads to form which products, CPC was carried out at −1.72 V in a CO2 saturated solution in the presence of phenylacetylene and the Ni(II) complex as is described in Section 2.2. At the end of the electrolysis, the solution was treated as described in Section 2. After separation of the main product, the spectral characteristics (Figs. S1–S3) indicated cinnamic acid has been formed with yield of approximately 70%. Comparing with other works, the product here is different [14,21–23,32,33]. The reason is that in the presence of the Ni(II) complex, the electrocatalytic reduction of CO2 occurs at less negative potential value (about 0.5 V). In the previous works [14,21–23,32,33], in the absence of any electron transfer mediator, CO2 is reduced at high overvoltage. Under the reported work conditions, phenylacetylene could also be reduced during the experiments. Therefore, it is logical to accept that there must be a different mechanism for the carboxylation process. Also, at the high overpotentials, the carboxylation of phenylacetylene cannot be limited to form a selective monocarboxylic acid [14,21–23,32,33]. In the present study, according to the voltammetric responses and also the spectral characteristics of the main isolated product, which is cinnamic acid, a mechanism is suggested as shown in Scheme 2. According to this scheme, the radical addition of CO2 ˙− to carbon 1 of phenylacetylene occurs at the first step and a new anion radical, Ph-C˙ CH˗COO−, is produced (Scheme 2, reaction (3)). The alternative product is Ph-C(COO−)CH˙ , which is formed from the anion radical addition of CO2 − to carbon 2 phenylacetylene (see Scheme 2). Since the delocalization of single electron, by the aromatic ring, in Ph-C˙CH(COO−) is more than that in Ph-C(COO−)CH˙ [34], it is expected that the radical addition CO2 ˙− to the carbon 1 of phenylacetylene is more probable [34]. Cathodic potential shift to less negative value in voltammogram (e) of Fig. 1 compared to voltammogram (d) of Fig. 1 is justified by the following chemical reaction (3), Scheme 2 [35], taking place after reactions (1) and (2). The product of reaction (3) takes up a proton [36] to produce the radical of carboxylic acid (reaction 4, Scheme 2). The hydronium ions are produced from the water impurity in the ACN solvent and Hofmann degradation of the supporting electrolyte [37,38] as discussed in Section 4.3. A comparison of voltammograms (d) and (e) of Fig. 1 indicates that the electrocatalytic peak current value of the complex in the presence of CO2 by itself (voltammogram d) is less than that observed in the presence of CO2 and phenylacetylene together (voltammogram e). This increase in the cathodic current indicates that the other electrocatalytic reduction process occurs. It means that an electron transfer is occurred from reduced form of the complex (Ni(I) complex) to the intermediate of Ph-C˙CH-COOH (reaction (5), Scheme 2) to form Ph-C−CH-COOH. Thus, it is expected the cathodic peak current value of voltammogram (e) of Fig. 1 is more than that of voltammogram (d) of Fig. 1. Finally, cinnamic acid is formed via taking up a proton by the produced anion (Scheme 2, reaction (6)). On the basis of the above discussion, as it is shown in Scheme 2, an EC′CCC′C mechanism is proposed for electrochemical synthesis of cinnamic acid from the electrocatalytic reduction of CO2 by the complex and the reaction of CO2 ˙− with phenylacetylene.
3.3 The effect of reaction conditions in selectivity of electrosynthesis products
Addition of CO2 to alkynes in one-compartment cells by using ordinary counter electrodes like platinum leads to poor selectivity and yield. However, electrocarboxylation by the use of sacrificial anodes in one-compartment cells gives better yields [19–23,33,39,40]. In fact, use of sacrificial anodes prevents formation of unwanted products by the oxidation of sacrificial anodes such as Mg [19–23,33,39,40]. Furthermore, oxidation of magnesium electrode in the anodic process leads to release magnesium ions into the electrolyte solution (reaction (1)). Then, precipitation of magnesium perchlorate is formed based on reaction (2):
Mg → Mg2+ + 2e | (1) |
(2) |
The formation of magnesium perchlorate during the electrolysis was proved by IR spectrum of precipitate (see Fig. S4 of Supplementary Information). In reaction (2), anion of the supporting electrolyte consumed and its cation (tetrabutylammonium cation, (C4H9)4N+) participate in the Hofmann degradation [37,38] (reaction (3)) to produce the hydronium ions, which is necessary for reactions (4) and (6) of Scheme 2.
(C4H9)4N+ → (C4H9)3N + C4H8 + H+ | (3) |
It is noted that the anion and radical anion that are produced from the cathodic process (Ph-C˙CH-COO− and Ph-C−CH(COOH) in Scheme 2) have the basic properties. These basic anions act as driving force for the Hofmann degradation of tetrabutylammonium cations [37,38].
4 Conclusion
Cinnamic acid is the main product of the coupling reaction of phenylacetylene and electrocatalytic activated CO2, CO2 ˙−, in the presence of the [NiII(Me4-NO2Bzo[15]tetraeneN4)] complex. The potential for electrocarboxylation of phenylacetylene in the presence of this complex is −1.72 V versus the reference electrode. In this potential, phenylacetylene cannot be reduced even in the presence of the Ni(II) complex. According to these results and also voltammetric responses, the most possible mechanism for such process is addition of CO2 − to phenylacetylene to form a mediator, which can take the second electron from the reduced form of the electrocatalyst (Ni(I) complex). This mechanism is supported by these facts: (1) increase in the catalytic current and also shift in the cathodic peak potential to less negative potentials after the addition of phenylacetylene to the solution of the Ni(II) complex that saturated with CO2 and (2) formation of cinnamic acid, in control potential coulometry, as the main product, which was characterized by FTIR, 1H NMR, and 13C NMR.