Transition metal oxides characterized by a triangular geometry of their metallic sublattice have received considerable attention because of their complex magnetic behavior. However, the ability of these lattices to generate properties in view of applications has not been fully developed as compared with square-like lattices such as those of perovskites, which are used as ferroelectrics, high TC superconductors, magnets, or colossal magnetoresistive materials. We report herein on recent studies of two classes of oxides with attractive properties either as multiferroics or as low-dimensional magnets.
1 Swedenborgite CaBaCo4O7 family: promising ferrimagnetic ferroelectrics
In the first class of oxides, the metallic cations form MO6 octahedra or MO4 tetrahedra and are displayed generally on a three-dimensional (3D) Mn sublattice built up of M4 tetrahedra and M3 triangles. This configuration induces geometric magnetic frustration, as shown in the numerous studies of the pyrochlore oxide family [1], and in a more general way produces frustrated magnets such as in kagome lattices of the spinel structure and in garnets [2–4].
Recently, complex magnetic transitions correlated to structural and/or electric transitions were observed and studied in pure tetrahedral kagome frameworks of the swedenborgite-type “114” LnBaCo407 cobaltates [5–21]. The room temperature hexagonal P31c structure of these oxides (Fig. 1a) consists of a 3D framework of corner-shared CoO4 tetrahedra, built up of two sorts of layers, kagome layers (K) and triangular layers (T) as shown in Fig. 2. As a consequence, the metallic [Co4] sublattice of these oxides is built up of “Co5” bitetrahedra interconnected through “Co3” triangles (Fig. 3). It results in a 2D geometric frustration in the (001)H plane, which strongly competes with the 1D magnetic ordering along c in the original hexagonal cell. Thus, these oxides have been considered as a new class of frustrated magnets, which out-of-plane magnetic coupling acts as an external magnetic field with respect to the in-plane 2D magnetic frustration [15].
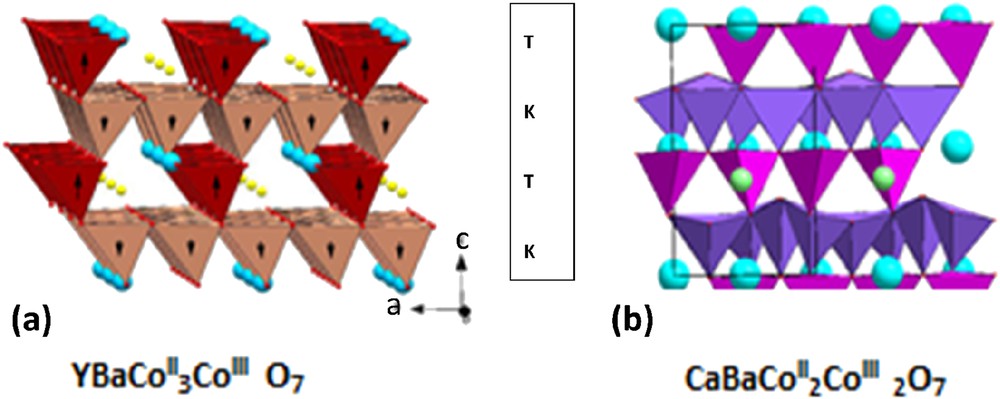
Structure of the “114” cobalt oxides: (a) hexagonal LnBaCo4O7 and (b) CaBaCo4O7. Both consist of the stacking along c of triangular (T) and kagome (K) layers.
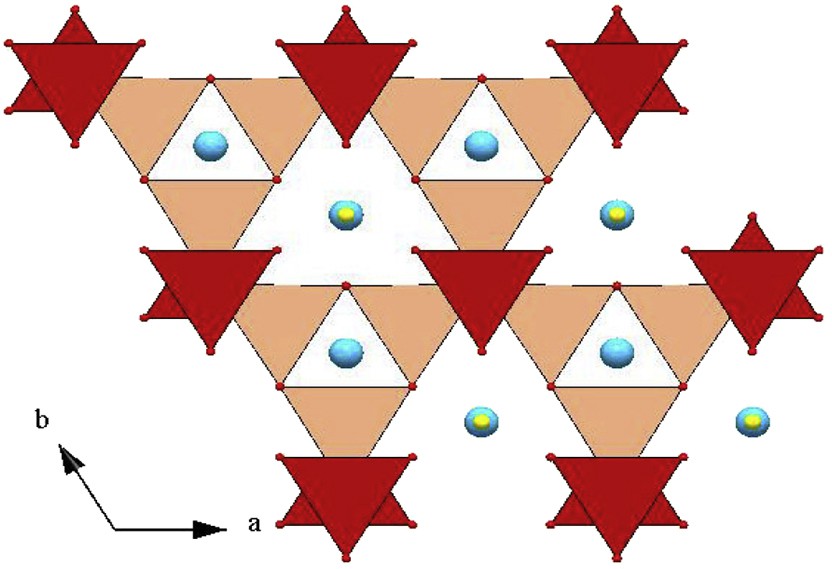
View of the structure along c of the triangular layers (T) as isolated CoO4 tetrahedra (red) and of the kagome layers (K) as corner-shared CoO4 tetrahedra (pink) in the “114” cobalt oxides.
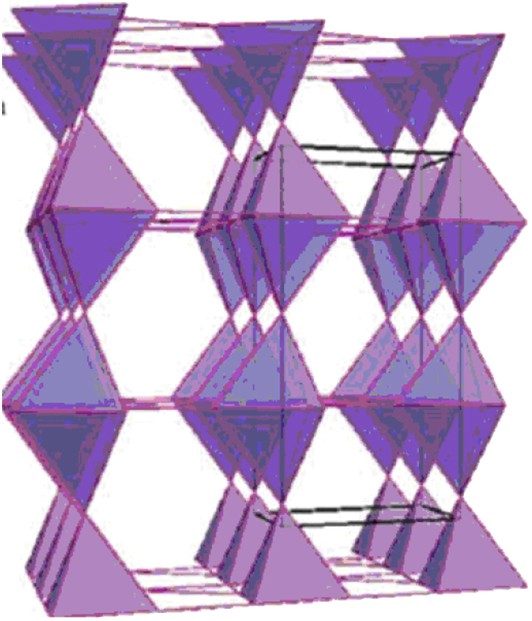
Cobalt [Co4] sublattice of hexagonal LnBaCo4O7 and orthorhombic CaBaCo4O7 oxides.
To modify the competition between this geometric 2D frustration and 1D magnetic ordering the possibility to replace the trivalent lanthanide by a divalent cation, calcium, was considered. The synthesis of the cobaltate CaBaCo4O7 [22,23] allows the mixed valence Co2+/Co3+ to be strongly modified, with an increase in the Co3+ content up to 50% with respect to LnBaCo4O7. This leads to a dramatic change in both structural and magnetic properties. Although closely related to LnBaCo4O7 oxides, the structure of CaBaCo4O7 (Fig. 1b) differs by a strong buckling of the kagome layers, the triangular layers remaining flat [23]. The first important structural character of this oxide deals with the very large orthorhombic distortion of its cell, which lifts the geometric frustration. It results in the appearance of charge and magnetic ordering. A complex ferrimagnetic structure is indeed observed at less than 64 K [23] (Fig. 4): it consists of ferromagnetic Co2+ zigzag chains, which are antiferromagnetically coupled with isolated mixed valent cobalt species Co3+/Co2+3d6/3d7L. The second remarkable feature concerns the fact that the structure of CaBaCo4O7 is noncentrosymmetric, with the Pbn21 space group, a necessary condition for the coexistence of ferroelectricity and ferrimagnetism, for the realization of multiferroic properties.
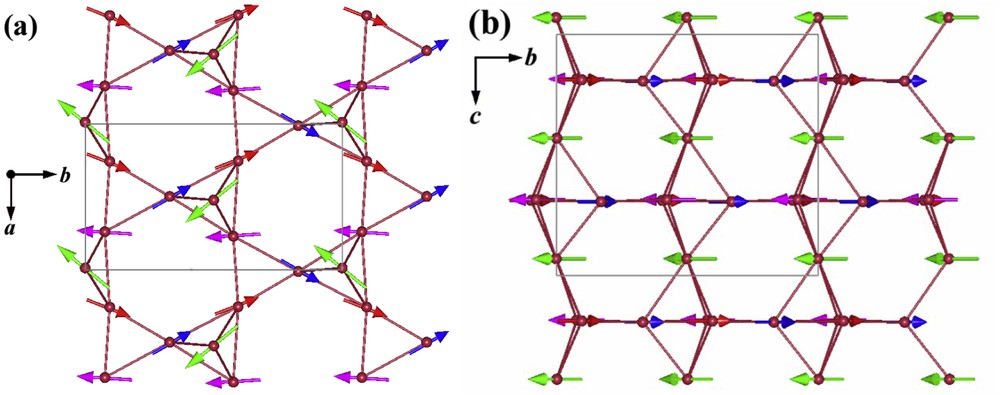
Ferrimagnetic structure of CaBaCo4O7: (a) Co2+ zigzag chains running along b (red and blue arrows) are antiferromagnetically coupled with isolated mixed valent cobalt Co3+/Co2+3d6/3d7L (green and pink arrows) and (b) bitetrahedral cobalt chains running along c.
On the basis of the aforementioned considerations, the dielectric properties of this cobaltate were first investigated on polycrystalline samples [24,25]. The dielectric permittivity and loss curves versus temperature measured at 100 kHz on these samples clearly show a peak at the same temperature as the ferrimagnetic transition TC = 64 K (Fig. 5a), which was interpreted as spin–charge coupling. Measurements of the electric polarization versus temperature with an electric field of 111 kV/m (Fig. 5b) performed on the same polycrystalline samples evidence also a transition around TC and importantly a sign change in the polarization by reversing the electric field. This behavior was then attributed to spin-driven ferroelectricity [24,25]. The study of large crystals of CaBaCo4O7 allowed us to confirm the multiferroic character of this oxide and showed that it is a remarkable magnetoelectric material [26] (Fig. 6). One indeed observes a sharp transition of the magnetization along b at 64 K, which coincides perfectly with the sharp peak of ε′ measured along c. Importantly, this oxide exhibits a gigantic variation in its induced electric polarization along c, ΔP = 17,000 μC/m2, a value never reached for magnetically induced ferroelectric polarization in multiferroic oxides, six times larger than that obtained for the perovskite CaMn7O12 [27,28]. However, Caignaert et al. could not observe switching of the electric polarization by reversing the electrical field for the crystal [26] in contrast to the polycrystalline samples [24,25].
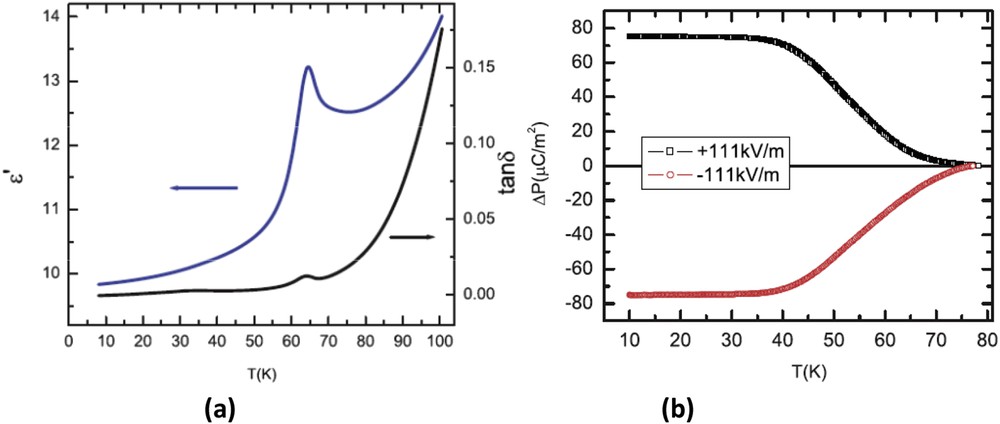
Polycrystalline CaBaCo4O7: (a) dielectric permittivity and loss vs T at 100 kHz and (b) electric polarization vs T showing a transition around TC with sign change of P by reversing E (111 kV/m).
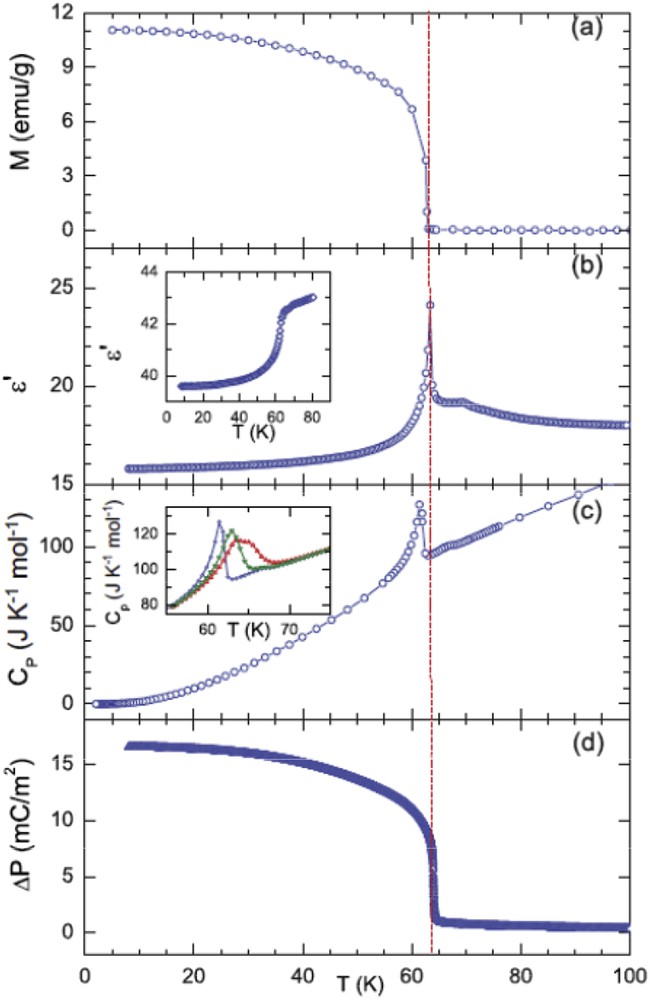
“Single” crystal data of CaBaCo4O7: (a) M(T)along b, (b) ε′(T) along c, (c) specific heat Cp(T), and (d) variation in polarization ΔP(T) along c.
From the latter results, Johnson et al. [29] reinvestigated the magnetoelectric coupling in CaBaCo4O7, using ab initio calculations and Landau theory; these authors showed that the observed giant variation in polarization is also compatible with exchange striction and that the strong magnetoelectric coupling close to TC originates from magnetoelastic coupling. From the fitting of their model with the crystal data extracted from Ref. [26], Johnson et al. concluded that CaBaCo4O7 is not a ferroelectric but a ferrimagnetic pyroelectric [29]. Unfortunately this latter study does not explain the switching behavior of the polarization observed on polycrystalline samples [24,25]. The recent investigation of this multiferroic by Fishman et al. [30] sheds light on this issue. Upon rotating a CaBaCo4O7 crystal about c in a fixed magnetic field applied along a, these authors showed, from the threefold splitting of the spin wave frequencies, that the latter is not a single crystal but a hexagonally twinned crystal. Magnetization measurements on crystals, combined with powder neutron and terahertz absorption spectroscopy allowed magnetic domains with a complex spin order in each domain to be evidenced [30]. Describing the cobalt framework as bitetrahedral chains parallel to c and ferrimagnetically coupled with each other in the ab plane Fishman et al. [30] concluded that the lifting of the geometric frustration in CaBaCo4O7 allows ferrimagnetism and ferroelectricity to coexist [30]. On the basis of the previously observed large changes in spin-induced polarization [26], these authors suggest that this oxide may have important technological applications, when a modest magnetic field less than 1 T is applied along b [30] close to TC. The high sensitivity of the magnetic properties of this swedenborgite to the doping of the cobalt sites with different cations such as Zn, Ga, Li, Al, Fe, and to the doping of Ba sites with Sr or Ln [31–39] offers many possibilities for tuning the characteristics of this multiferroic. The recent study of the ferroelectric Ni-doped CaBaCo4O7, which exhibits an enhanced ordering temperature at 82 K [40], supports this statement.
2 Spin chain oxides Sr4–xCaxMn2CoO9: toward low-dimensional magnets
The second class of oxides belongs to the triangular spin chain oxide family. Their hexagonal structure consists of single chains of face-sharing MO6 octahedra and trigonal prisms displayed at the corners of a triangular lattice. The Ca3Co2O6 cobaltate, whose [Co2O6] chains exhibit a 1:1 ordering of the CoO6 prisms and CoO6 octahedra (Fig. 7a), is the archetype of these spin-chain compounds. The latter was found to exhibit several original features [41–48] such as a strong Ising-like character due to antiferromagnetic interchain and ferromagnetic intrachain coupling, with magnetization plateaus, originating from the close energy values of the cobalt moments in the triangular planes perpendicular to the cobalt chains. The isotypic Ca3MnCoO6 compound [49] also characterized by a 1:1 intrachain ordering of the MnO6 octahedra and CoO6 prisms was revealed to be a multiferroic with complex magnetic behavior [50–53]. For these Ca3MM′O6 oxides, partially disordered antiferromagnetism was often reported to describe the magnetic interactions between the Ising chains on the triangular lattice [54–58].

Perspective view of the structure of the spin chain oxides (a) Ca3MnCoO6 and (b) Sr4Mn2CoO9. Note the 1:1 and 2:1 ordering of the MnO6 octahedra (rust colored) and CoO6 trigonal prisms (purple). Ca2+ and Sr2+ cations are omitted for the sake of clarity.
The oxides Sr4Mn2CoO9 [59] and Sr3CaMn2CoO9 [60] can be described as the second members of this spin chain oxide family: in this hexagonal structure (Fig. 7b), the [Mn2CoO9] chains are also formed of face-sharing MnO6 octahedra and CoO6 prisms, but a 2:1 ordering “Mn2Co” between the octahedra and the prisms within each chain is observed. The magnetism of the latter compounds has not been intensely studied in contrast to the first members, most probably because of the ill-defined glassy character of their magnetic properties. Indeed, Sr4Mn2CoO9 [59] was found to exhibit a behavior close to a spin glass, whereas the enhancement of magnetic correlations was observed for Sr3CaMn2CoO9 [60], but no long-range ordering could be reached. Bearing in mind that the interchain distances should significantly influence the magnetic interactions between the chains, the size effect of the Ca2+/Sr2+ cations was recently investigated in the oxides Sr4–xCaxMn2CoO9, by comparing the magnetic behavior of Sr4Mn2CoO9 (x = 0) and Sr2Ca2Mn2CoO9 (x = 1) [61]. The χ′(T) curves of these phases (Fig. 8) showed that they both exhibit a frequency-dependent bump around 6 K (labeled as regime 2 in Fig. 8), but differ in their behavior at higher temperature. Sr2Ca2Mn2CoO9 is characterized by a frequency-independent maximum of χ′ around 29 K (labeled as regime 3 in Fig. 8), whereas Sr4Mn2CoO9 displays a strongly dependent frequency peak around 10–15 K (labeled as regime 1). The regime 3 observed for Sr2Ca2Mn2CoO9 could easily be attributed to the existence of long-range antiferromagnetic ordering (LRO) with TN = 29 K in agreement with neutron diffraction data. The regime 1 observed for Sr4Mn2CoO9 and the regime 2 observed for both compounds were clearly identified from χ″(T) curves and from Argand plots that were shown to be consistent with the Cole–Cole model.
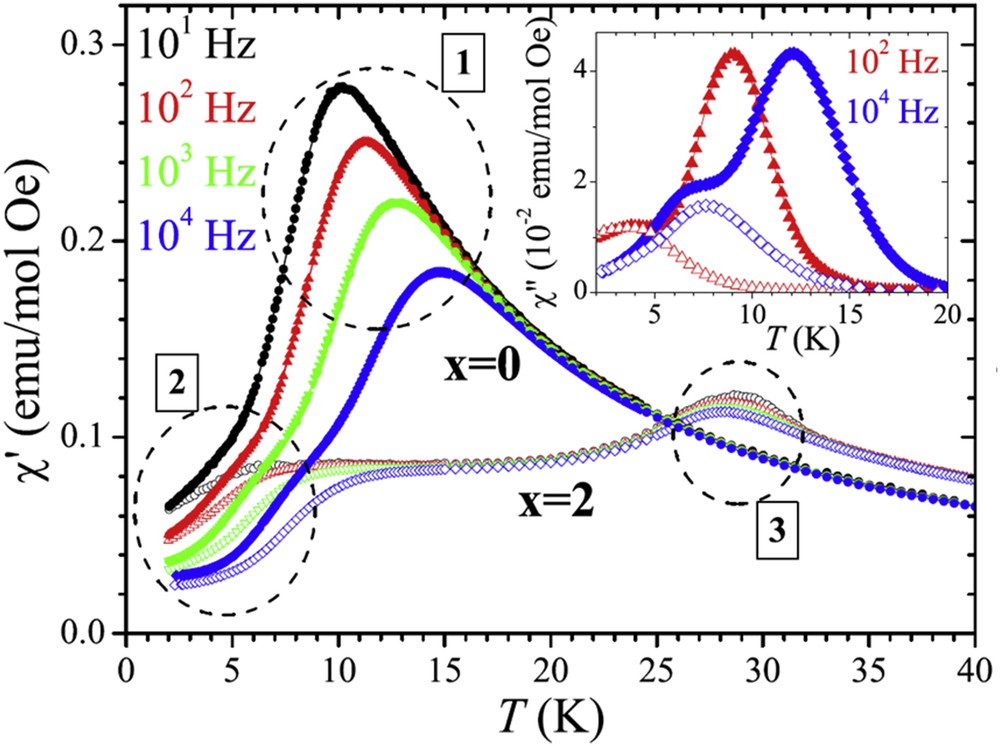
Sr4–xCaxMn2CoO9: in-phase (main panel) and out-of-phase (inset) susceptibility of x = 0 (filled symbols) and x = 2 (open symbols). The dotted circles highlight the three regimes labeled 1, 2, and 3.
The relaxation time curves τc (1/T) extracted from these investigations (Fig. 9) summarize these results. The temperature-independent character of τc observed for Sr2Ca2Mn2CoO9 (regime 3 in Fig. 9) originates from LRO. The Arrhenius-like evolution of τc versus 1/T observed for Sr4Mn2CoO9 (regime 1 in Fig. 9) rules out the possibility of a spin glass behavior for this compound and is similar to that of a single chain magnet (SCM). Moreover, the values of τ0 ∼ 10−11 s and of Δ ∼ 167 K obtained from the maxima in χ″(T), close to those reported for SCM molecular compounds containing Co2+ [62–64] confirm this statement. The genuine SCM behavior of this phase was then demonstrated further by χdc measurements and from M(H) hysteresis loops. In this way, it was shown that blocking effects associated with slow spin dynamics yield remanence and coercivity in the absence of LRO for Sr4Mn2CoO9 [61]. The τc (1/T) evolution of regime 3 (Fig. 9), similar for both oxides, which coexists with SCM in Sr4Mn2CoO9 and with LRO in Sr2Ca2Mn2CoO9 was attributed to the fact that Co2+ can act as a single ion magnet (SIM), in agreement with the behavior observed for SIM in Co2+ complexes [65–68].
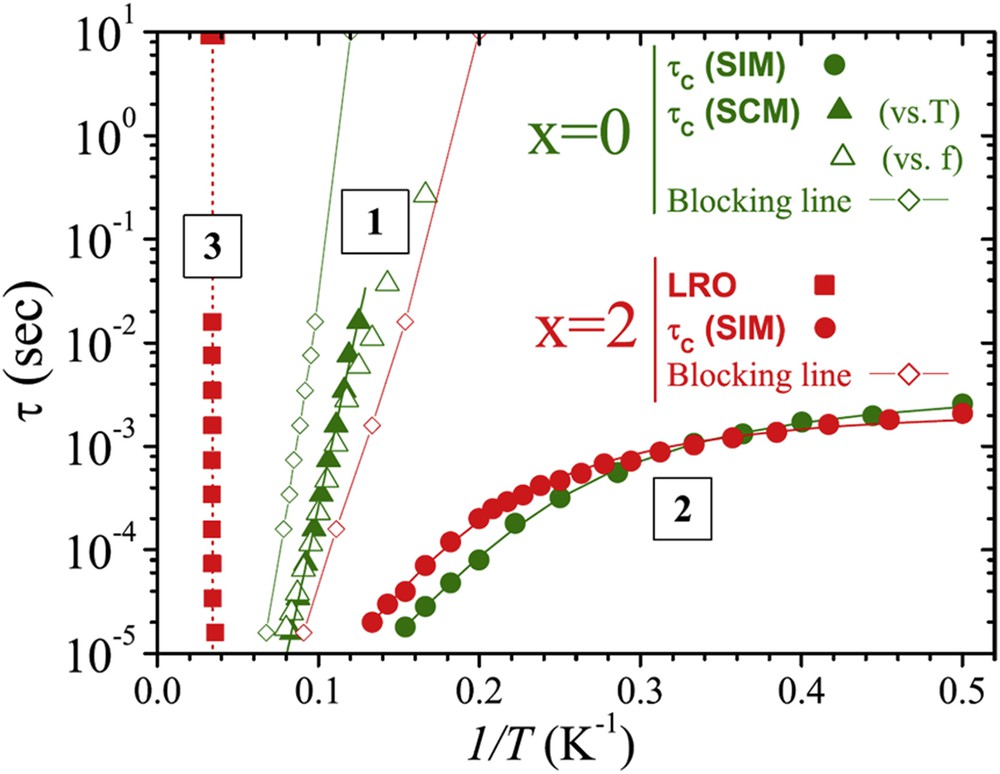
Sr4–xCaxMn2CoO9: Arrhenius plot of the median relaxation times of regime 1 (SCM) in x = 0 and regime 2 (SIM) in both compounds (x = 0 and 2), with the corresponding fitting lines. Regime 3, derived from the high T maxima of χdc (T) in x = 2 is ascribed to the onset of LRO. The open diamonds delineate the domains where χ(T) starts being affected by spin blocking effects, i.e., SCM in x = 0 and SIM in x = 2. Also shown are dc data (associated with a characteristic time of 10 s) corresponding to regime 3 (midpoint of spontaneous magnetization) and to the blocking lines [start of the drop in χdc(T) zero field cooled (ZFC)].
These observations of SCM and SIM behaviors in purely anhydrous inorganic materials are quite unique. They show the possibility to realize low-dimensional magnets (0D or 1D) similar to those obtained for the large family of molecular compounds, which have been extensively studied for the generation of single molecule magnets [69,70] and SCMs [62,71]. In these oxides and especially in Sr4Mn2CoO9, the Sr2+ cations because of their larger size allow the distances between the magnetic chains to be sufficiently increased to considerably weaken the 3D magnetism. In this way, they play the role of organic ligands or molecules that are generally used for shielding the magnetic interactions between the transition metal clusters or chains in molecular magnets. Bearing in mind that low-dimensional magnets have been the object of considerable interest for their potential applications in information storage [72,73] and in quantum computation [74], these oxides, which are highly stable as compared to molecular compounds, open the route for future investigations.
3 Conclusions
These recent studies show that transition metal oxides, which involve triangular metallic sublattices, exhibit besides magnetic frustration a great potential for the generation of materials with attractive properties in view of applications either as ferroelectric multiferroics or as low-dimensional magnets. Bearing in mind that the appearance of such properties stems from either the lifting of the geometric frustration or the weakening of chain interactions in triangular systems, this opens the route to the investigation of numerous alkaline earth-based transition metal oxides. In these systems the inter-related structural and chemical parameters such as distortion of the structure, valence of the transition element, oxygen stoichiometry, size of alkaline earth cation, and order–disorder phenomena have to be controlled carefully.